Teachable Moments | November 27, 2023
NASA Balloon Mission Designed to See the Space Between Stars
Get to know GUSTO and learn how to bring the science and engineering behind this unique balloon-based mission into the classroom.
A NASA balloon mission designed to study the interstellar medium – the space between stars – will take to the skies above Antarctica in December 2023.
Read on to learn how the GUSTO mission's unique design and science goals can serve as real-life examples of STEM concepts. Then, explore lessons and resources you can use to get students learning more.
What the GUSTO Mission Will Do
Though many people think of space as empty except for things like stars, planets, moons, asteroids, meteors, and comets, it’s anything but. Typically, there is one molecule of matter in every cubic centimeter of the space between stars known as the interstellar medium. In more dense clouds of interstellar gas, there could be as many as 1,000,000 molecules per cubic centimeter. It might not seem like much compared with the 10,000,000,000,000,000,000 molecules in every cubic centimeter of air we breathe, but the interstellar medium can tell us a lot about how stars and planets form and what role gases and dust play in our galaxy and others.

This diagram shows the life cycles of Sun-like and massive stars. Credit: NASA, Night Sky Network | › Learn more about star life cycles
Like plants and animals, stars have a life cycle that scientists want to better understand. Gases and dust grains that make up a dense interstellar cloud, known as a nebula, can become disturbed, and under the pull of their own gravity, begin collapsing in on themselves. Eventually stars form from the gas and planets form from the dust. As a star goes through its life, it eventually runs out of sources of energy. When this happens, the star dies, expelling gases – sometimes violently, as in a supernova – into a new gas cloud. From here, the cycle can start again. Scientists want to know more about the many factors at play in this cycle. This is where GUSTO comes in.
GUSTO – short for Galactic/Extragalactic ULDB Spectroscopic Terahertz Observatory – is a balloon-based telescope that will study the interstellar medium, the small amount of gas and dust between the stars. From its vantage point high above almost all of the Earth’s atmosphere, GUSTO will measure carbon, nitrogen, and oxygen emissions in the far-infrared portion of the electromagnetic spectrum, focusing its sights on the Milky Way galaxy and the nearby galaxy known as the Large Magellanic Cloud.

Our galaxy, the Milky Way, has hundreds of billions of stars and enough gas and dust to make billions more stars. Credit: NASA | › Full image and caption
The mission is designed to provide scientists with data that will help them understand the complete lifecycle of the gas and dust that forms planets and stars. To achieve its goals, GUSTO will study:
- The composition and formation of molecular clouds in these regions.
- The formation, birth, and evolution of stars from molecular clouds.
- The formation of gas clouds following the deaths of stars. And the re-start of this cycle.
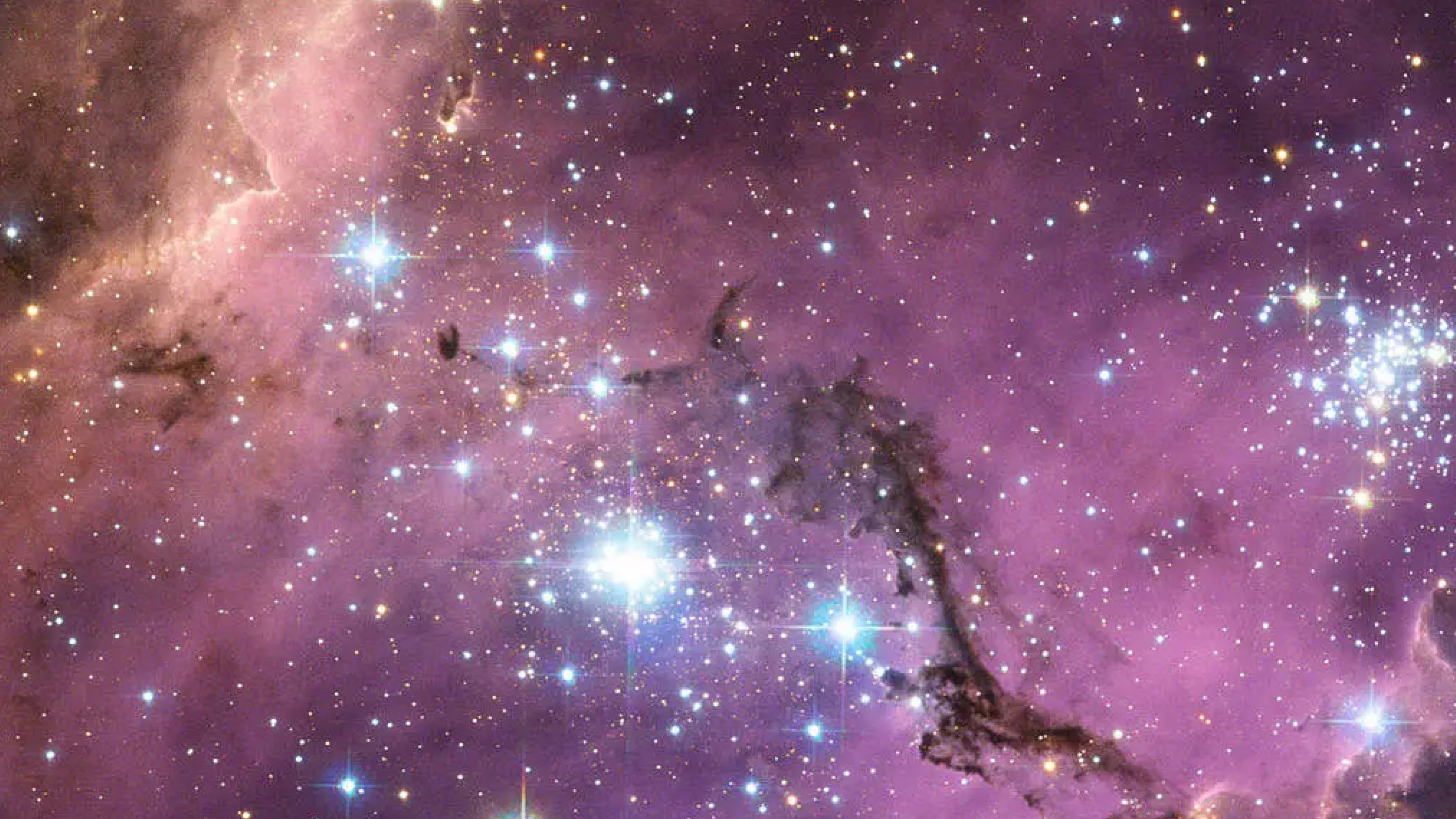
Nearly 200,000 light-years from Earth, the Large Magellanic Cloud is a satellite galaxy of the Milky Way. Vast clouds of gas within it slowly collapse to form new stars. In turn, these light up the gas clouds in a riot of colors, visible in this image from the Hubble Space Telescope. Credit: NASA | › Full image and caption
Scientists hope to use the information collected by GUSTO to develop models of the Milky Way and Large Magellanic Cloud. Studying these two galaxies allows scientists to observe more details and make more accurate models. Those models can then be used for comparing and studying more distant galaxies that are harder to observe.
Why Fly on a Balloon?
Unlike most NASA missions, GUSTO won’t launch on a rocket. It will be carried to approximately 120,000 feet (36.5 kilometers) above Antarctica using what’s known as a Long Duration Balloon, or LDB.
Balloon missions provide a number of advantages to scientists conducting research. They are more affordable than missions that go to space and require less time to develop. They also offer a way to test new scientific instruments and technologies before they are used in space. For these reasons, balloons have become a popular way for university students to gain experience building and testing science instruments.
Explore how balloons are being used for Earth and space science in this video from the Johns Hopkins Applied Physics Laboratory, which is providing the mission operations for GUSTO and the balloon gondola where the mission's instruments will be mounted. | Watch on YouTube
GUSTO's use of the Long Duration Balloon provided by NASA’s Balloon Science Program offers several advantages over other types of scientific balloons. Conventional scientific balloons stay aloft for a few hours or a few days and rely on the balloon maintaining a line-of-sight to send and receive data. Long Duration Balloons use satellites for sending data and receiving commands and can stay afloat for a few weeks to a couple of months.
Made with a thin, strong, plastic film called polyethylene, LDBs are partially inflated with helium. As the balloon rises, the surrounding air pressure decreases, allowing the gas inside the balloon to expand, increasing the volume and pressure of the balloon. When fully expanded, the balloon has a volume of around 40 million cubic feet (1.1 million cubic meters). That’s big enough to fit an entire football stadium inside.

GUSTO will be attached to a balloon gondola like the one depicted in this artist's rendering. | + Expand image
The telescope itself will be attached to a platform known as a gondola, which is home to several components that make the mission possible. The multi-axis control system will keep the platform stable during flight, allowing for precisely pointing GUSTO’s 35-inch (90-centimeter) diameter telescope in the right direction. Cryocoolers and liquid helium will keep the telescope’s scientific instruments at the necessary low temperature of -452°F (4° Kelvin). And the gondola will house a radio system that allows operators on the surface to control the balloon and telescope. All these systems will be powered by lithium-ion batteries charged during flight by a set of solar arrays.
Location is Everything
GUSTO is designed to measure terahertz wavelengths (in the far-infrared portion of the electromagnetic spectrum), a range of energy that is easily absorbed by water vapor. However, the observatory's altitude will put it in the upper half of the stratosphere and above 99% of the water vapor in the atmosphere. This makes it an ideal location for the mission to make its measurements and avoid factors that might otherwise obstruct its view.

GUSTO will make its observations from the upper half of the stratosphere, which offers several benefits over observing from lower in the atmosphere or from the ground. Credit: NASA | › Explore the interactive graphic
The stratosphere offers another advantage for GUSTO. This layer of the atmosphere warms as altitude increases, making the top of the stratosphere warmer than the bottom. The colder air at the bottom and warmer air at the top prevents mixing and air turbulence, making the air very stable and providing a great place to observe space. You may have noticed this stability if you’ve seen a flat-topped anvil-shaped storm cloud. That flat top is the cloud reaching the bottom of the stratosphere, where the stable air prevents the cloud from mixing upward.
But why fly GUSTO above Antarctica? Even though balloons can be launched from all over the planet, the 24 hours of sunlight per day provided by the Antarctic summer make the south polar region an ideal launch location for a solar-powered mission like GUSTO. But more important is a weather phenomenon known as an anticyclone. This weather system is an upper-atmosphere counter-clockwise wind flow that circles the South Pole about every two weeks. The Antarctic anticyclone allows for long balloon flights of missions that can be recovered and potentially reflown.
Preparing for Liftoff
To launch a balloon mission in Antarctica, weather conditions have to be just right. The anticyclone typically forms in mid-December but can arrive a little earlier or a little later. Even with the anticyclone started, winds on the ground and in the first few hundred feet of the atmosphere need to be under six knots (seven miles per hour) for GUSTO to launch. A NASA meteorologist provides daily updates on the cyclone and the ground.
Once weather conditions are good and the balloon is launched, it will circle Antarctica about once every 14 days with the wind. The anticyclone typically lasts one to two months. Because GUSTO may be in the air for more than two months, it’s possible that the mission will continue after the anticyclone ends, causing the balloon to drift northward as winter progresses.
Bring GUSTO Into the Classroom
The GUSTO mission is a great opportunity to engage students with hands-on learning opportunities. Students can build a planetary exploration balloon and model how interstellar dust forms into planets. Explore these lessons and resources to get students excited about the STEM involved in the mission.
Resources for Educators
- Lesson
Make a Planetary Exploration Balloon
In this engineering challenge, students must stay within design limitations while creating a balloon and gondola system that can descend or ascend at a given rate or maintain its altitude.
Subject Science
Grades 3-12
Time Less than 30 mins
- Lesson
The Science of Color
Quickly and easily model how colors reflect, absorb, and interact with each other in the classroom or online using your computer’s camera.
Subject Science
Grades 2-8
Time 30-60 mins
- Interactive
Star Formation: Eagle Nebula
View the Eagle Nebula in different wavelengths to see how new details emerge.
Subject Science
Resources for Students
- Project
Make a Planetary Exploration Balloon
Find out how NASA uses balloons to explore Earth and space and then take on a challenge to design your own balloon explorer inspired by what you've learned!
Subject Science
Grades 3-12
Time 30-60 mins
- Article
What Is a Galaxy?
Learn what galaxies are made of in this article from NASA Space Place.
- Article
How Old Are Galaxies?
Get the answer in this article from NASA Space Place.
- Interactive
Explore the Electromagnetic Spectrum
Click through this interactive from NASA Space Place all about the electromagnetic spectrum.
NASA's Universe of Learning materials are based upon work supported by NASA under award number NNX16AC65A to the Space Telescope Science Institute, working in partnership with Caltech/IPAC, Center for Astrophysics | Harvard & Smithsonian, and the Jet Propulsion Laboratory.
TAGS: GUSTO, Astronomy, Astrophysics, Science, Teaching, Learning, K-12, Classroom, Teachable Moments, Universe of Learning, Balloon Mission, Missions
Teachable Moments | July 24, 2023
Exploring the Mystery of Our Expanding Universe
Learn about a new mission seeking to understand some of the greatest mysteries of our universe, and explore hands-on teaching resources that bring it all down to Earth.
Scientists may soon uncover new insights about some of the most mysterious phenomena in our universe with the help of the newly launched Euclid mission. Built and managed by the European Space Agency, Euclid will use a suite of instruments developed, in part, by NASA's Jet Propulsion Laboratory to explore the curious nature of dark energy and dark matter along with their role in the expansion and acceleration of our universe.
Read on to learn how the Euclid mission will probe these cosmological mysteries. Then, find out how to use demonstrations and models to help learners grasp these big ideas.
Why It’s Important
No greater question in our universe promotes wonder in scientists and non-scientists alike than that of the origin of our universe. The Euclid mission will allow scientists to study the nearly imperceptible cosmic components that may hold exciting answers to this question.
Edwin Hubble's observations of the expanding universe in the 1920s marked the beginnings of what's now known as the big-bang theory. We've since made monumental strides in determining when and how the big bang would have taken place by looking at what's known as cosmic background radiation using instruments such as COBE and WMAP in 1989 and 2001, respectively. However, there's one piece of Hubble's discovery that still has scientists stumped: our universe is not only expanding, but as scientists discovered in 1998, that expansion is also accelerating.
This side by side comparison shows a constant rate of expansion of the universe, represented by the expanding sphere on the left, and an accelerating rate of expansion of the universe, represented by the expanding sphere on the right. Each dot on the spheres represents a galaxy and shows how galaxies move apart from each other faster in the universe that has an accelerating rate of expansion. | Watch on YouTube
How can this be? It makes intuitive sense that, regardless of the immense force of the big bang that launched all matter across the known universe 13.8 billion years ago, that matter would eventually come to a rest and possibly even start to collapse. Instead, it's as if we've dropped a glass onto the ground and discovered that the shards are flying away from us faster and faster into perpetuity.
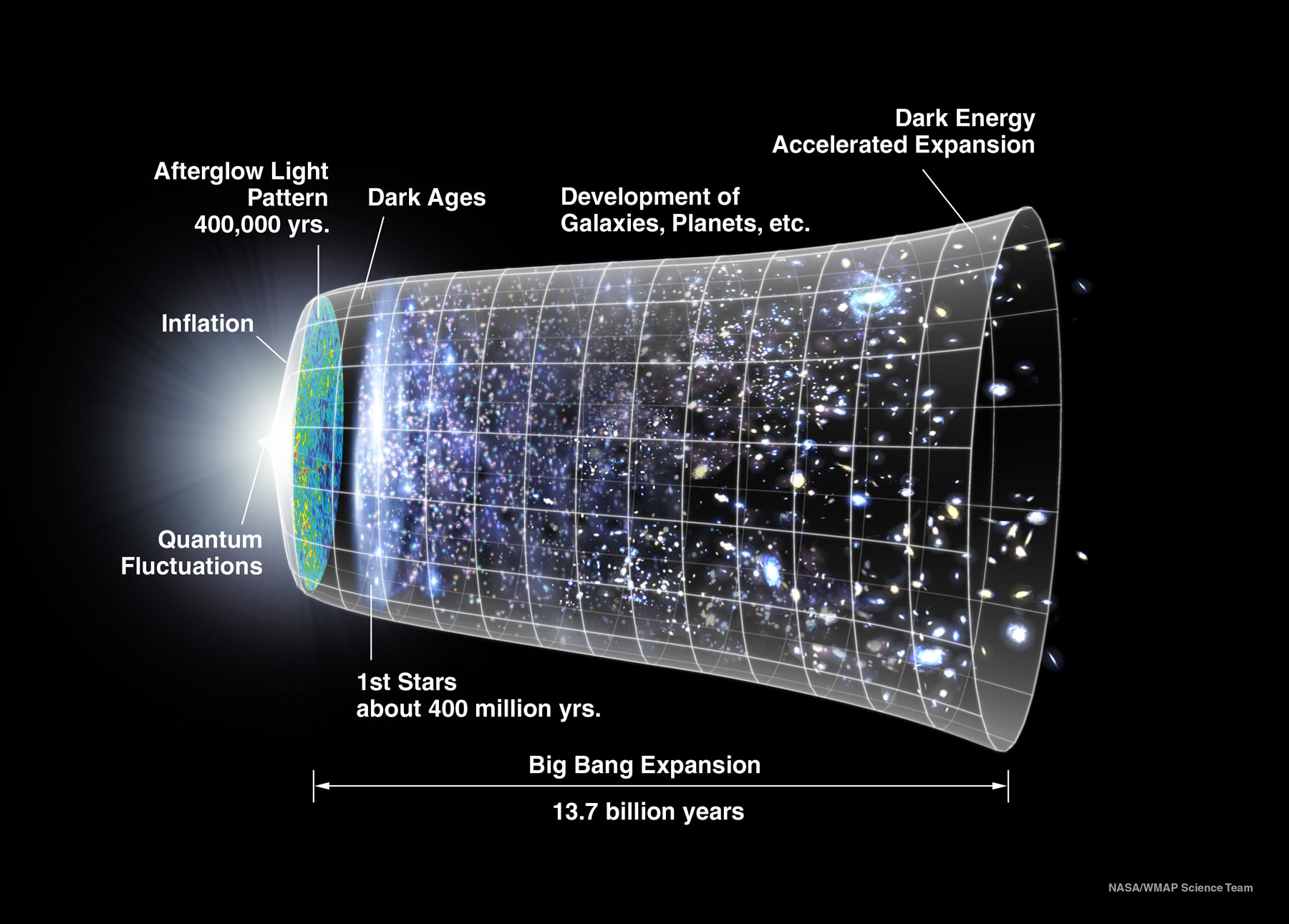
An illustrated timeline of the universe. Credit: WMAP | + Expand image
Scientists believe that answers may lie in two yet-to-be-understood factors of our universe: dark matter and dark energy. Dark matter is unlike the known matter we experience here on Earth, such as what's found on the periodic table. We can't actually see dark matter; we can only infer its presence. It has mass and therefore gravity, making it an attractive force capable of pulling things together. Amazingly, dark matter makes up roughly 27% of the known universe compared with the much more modest 5% of "normal matter" that we experience day to day. However, dark matter is extremely dilute throughout the universe with concentrations of 105 particles per cubic meter.
This animated pie chart shows rounded values for the three known components of the universe: visible matter (5%), dark matter (27%), and dark energy (68%). Credit: NASA's Goddard Space Flight Center | › Full video and caption
In opposition to the attractive force of dark matter, we have dark energy. Dark energy is a repulsive force and makes up roughly 68% of energy in the known universe. Scientists believe that the existence of dark energy and the amount of repulsion it displays compared with dark matter is what's causing our universe to not only expand, but also to expand faster and faster.
Dr. Jennifer Wiseman, a senior project scientist with the Hubble Space Telescope mission, explains how the mission has been helping scientists learn more about dark energy. Credit: NASA Goddard | Watch on YouTube
But to truly understand this mysterious force and how it interacts with both dark matter and normal matter, scientists will have to map barely detectable distortions of light traversing the universe, carefully measuring how that light changes over time and distance in every direction. As JPL Astrophysicist Jason Rhodes explains, “Dark energy has such a subtle effect that we need to survey billions of galaxies to adequately map it.”
And that's where Euclid comes in.
How It Works
The European Space Agency and NASA each contributed to the development of the Euclid mission, which launched from Cape Canaveral Space Force Station in Florida on July 1. The spacecraft consists of a 1.2-meter (48-inch) space telescope and two science instruments: an optical camera and a near-infrared camera that also serves as a spectrometer. These instruments will provide a treasure trove of data for scientists of numerous disciplines, ranging from exoplanet hunters to cosmologists.

Light waves get stretched as the universe expands similar to how this ink mark stretches out as the elastic is pulled. Get students modeling and exploring this effect with this standards-aligned math lesson. Credit: NASA/JPL-Caltech | + Expand image
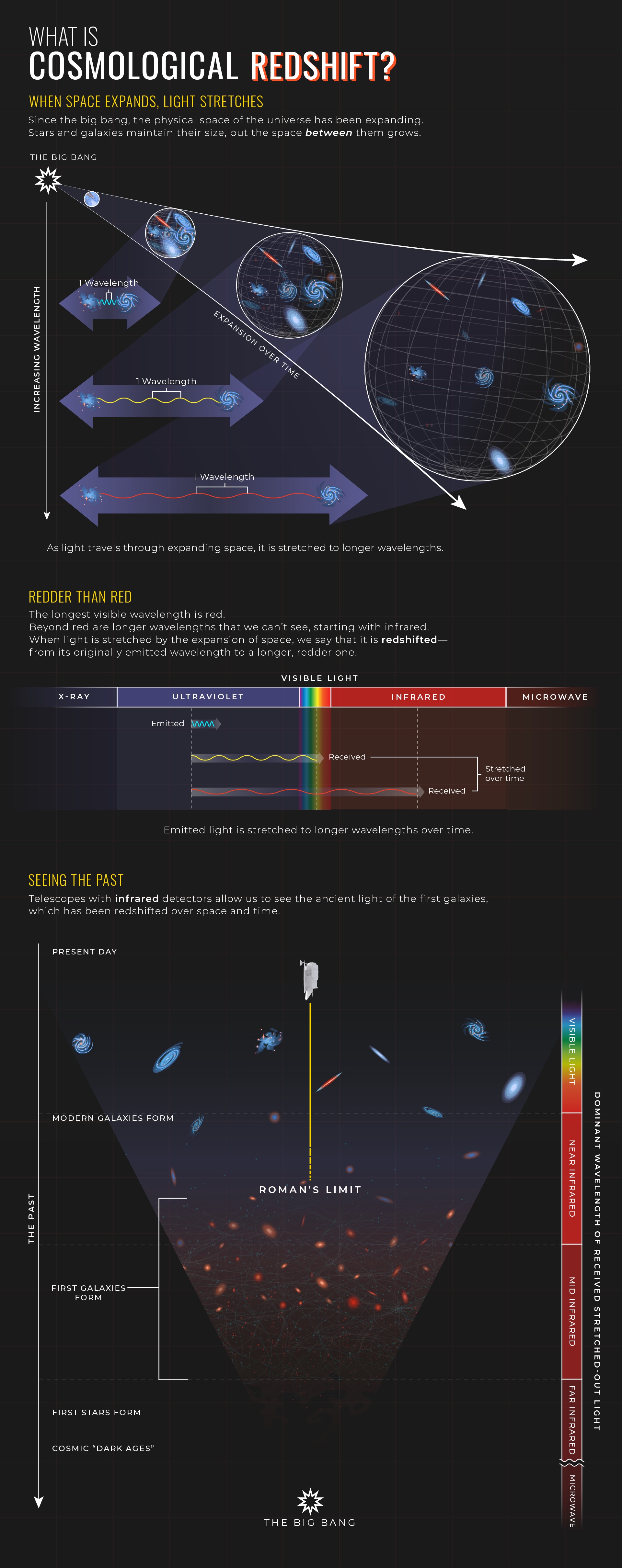
This graphic illustrates how cosmological redshift works and how it offers information about the universe’s evolution. Credit: NASA, ESA, Leah Hustak (STScI) | › Full image and caption
As Gisella de Rosa at the Space Telescope Science Institute explains, “The ancillary science topics we will be able to study with Euclid range from the evolution of the objects we see in the sky today to detecting populations of galaxies and creating catalogs for astronomers. The data will serve the entire space community.”
The cameras aboard Euclid will operate at 530-920 nanometers (optical light) and at 920-2020 nanometers (near infrared) with each boasting more than 576 million and 65 million pixels, respectively. These cameras are capable of measuring the subtle changes to the light collected from celestial objects and can determine the distances to billions of galaxies across a survey of 15,000 square degrees – one-third of the entire sky.
Meanwhile, Euclid's spectrometer will collect even more detailed measurements of the distance to tens of millions of galaxies by looking at redshift. Redshift describes how wavelengths of light change ever so slightly as objects move away from us. It is a critical phenomenon for measuring the speed at which our universe is expanding. Similar to the way sound waves change as a result of the Doppler effect, wavelengths of light are compressed to shorter wavelengths (bluer) as something approaches you and extended to longer wavelengths (redder) as it moves away from you. As determined by a Nobel Prize winning team of astronomers, our universe isn’t just red-shifting over time, distant objects are becoming redder faster.
Euclid will measure these incredibly minuscule changes in wavelength for objects near and far, providing an accurate measurement of how the light has changed as a factor of time and distance and giving us a rate of acceleration of the universe. Furthermore, Euclid will be able to map the relative densities of dark matter and normal matter as they interact with dark energy, creating unevenly distributed pockets of more attractive forces. This will allow scientists to identify minute differences in where the universe is expanding by looking at the way that light is altered or "lensed."
The multi-dimensional maps created by Euclid – which will include depth and time in addition to the height and width of the sky – will inform a complementary mission already in development by NASA, the Nancy Grace Roman Space Telescope. Launching in 2026, this space telescope will look back in time with even greater detail, targeting areas of interest provided by Euclid. The telescope will use instruments with higher sensitivity and spatial resolution to peer deeper into redshifted and faint galaxies, building on the work of Euclid to look farther into the accelerating universe. As Caltech’s Gordon Squires describes it: “We’re trying to understand 90% of our entire universe. Both of these telescopes will provide essential data that will help us start to uncover these colossal mysteries.”
Teach It
The abstract concepts of the scope and origin of our universe and the unimaginable scale of cosmology can be difficult to communicate to learners. However, simple models and simulations can help make these topics more tangible. See below to find out how, plus explore more resources about our expanding universe.Resources
- Educator Guide
Model the Expanding Universe
Students learn about the role of dark energy and dark matter in the expansion of the universe, then make a model using balloons.
Subject Science
Grades 6-12
Time 30-60 mins
- Educator Guide
How Do We See Dark Matter?
Students will make observations of two containers and identify differences in content, justify their claims and make comparisons to dark matter observations.
Subject Science
Grades 6-12
Time Less than 30 mins
- Educator Guide
Math of the Expanding Universe
Students will learn about the expanding universe and the redshift of lightwaves, then perform their own calculations with a distant supernova.
Subject Science
Grades 9-12
Time 30-60 mins
- Collection
ViewSpace: Dark Energy Videos
Use this collection of short videos to introduce learners to the concept of dark energy.
- Collection
ViewSpace: Dark Matter Videos
Use this collection of short videos to introduce learners to the concept of dark matter.
- Science Briefing
Hubble Constant Discrepancies: Implications for Our Expanding Universe
Hear experts discuss how the rate of the universe is measured in different cosmological epochs and what the differences in those measures can tell us.
- Game
Roman Space Observer
How many astrophysical objects can you catch?
Explore More
- Article for Kids: What Is a Supernova?
- Article for Kids: What Is Dark Matter?
- Article: What Is Dark Energy?
- Article: Gravitational Lensing - Shining a Light on Dark Matter
- Facts & Figures: Euclid Mission - NASA
- Facts & Figures: Nancy Grace Roman Space Telescope
NASA's Universe of Learning materials are based upon work supported by NASA under award number NNX16AC65A to the Space Telescope Science Institute, working in partnership with Caltech/IPAC, Center for Astrophysics | Harvard & Smithsonian, and the Jet Propulsion Laboratory.
TAGS: K-12 Education, Teaching, Teachers, Educators, Resources, Universe, Dark Matter, Dark Energy, Euclid, Nancy Grace Roman Space Telescope, Universe of Learning
Teachable Moments | October 24, 2022
X-Ray Vision and Polarized Glasses Unite to Uncover Mysteries of the Universe
A NASA space telescope mission is giving astronomers a whole new way to peer into the universe, allowing us to uncover long-standing mysteries surrounding objects such as black holes. Find out how it works and how to engage students in the science behind the mission.
Some of the wildest, most exciting features of our universe – from black holes to neutron stars – remain mysteries to us. What we do know is that because of their extreme environments, some of these emit highly energetic X-ray light, which we can detect despite the vast distances between us and the source.
Now, a NASA space telescope mission is using new techniques to not only scout out these distant phenomena, but also provide new information about their origins. Read on to learn how scientists are getting exciting new perspectives on our universe and what the future of X-ray astronomy holds.
How They Did It
In 2021, NASA launched the Imaging X-Ray Polarimeter Explorer, or IXPE, through a collaboration with Ball Aerospace and the Italian Space Agency. The space telescope is designed to operate for two years, detecting X-rays emitted from highly energetic objects in space, such as black holes, different types of neutron stars (e.g., pulsars and magnetars) and active galactic nuclei. In its first year, the telescope is focusing on roughly a dozen previously studied X-ray sources, spending hours or even days observing each target to reveal new data made possible by spacecraft's scientific instruments.
IXPE isn't the first telescope to observe the universe in X-ray light. NASA's Chandra X-ray Observatory, launched in 1999, has famously spent more than 20 years photographing our universe at a wavelength of light exclusively found in high-energy environments, such as where cosmic materials are heated to millions of degrees as a result of intense magnetic fields or extreme gravity.
Using Chandra, scientists can assign colors to the different energy levels, or wavelengths, produced by these environments. This allows us to get a picture of the highly energetic light ejected by black holes and tiny neutron stars – small, but extremely dense stars with masses 10-25 times that of our Sun. These beautiful images, such as from Chandra’s first target, Cassiopeia A (Cas A for short), show the violent beauty of stars exploding.
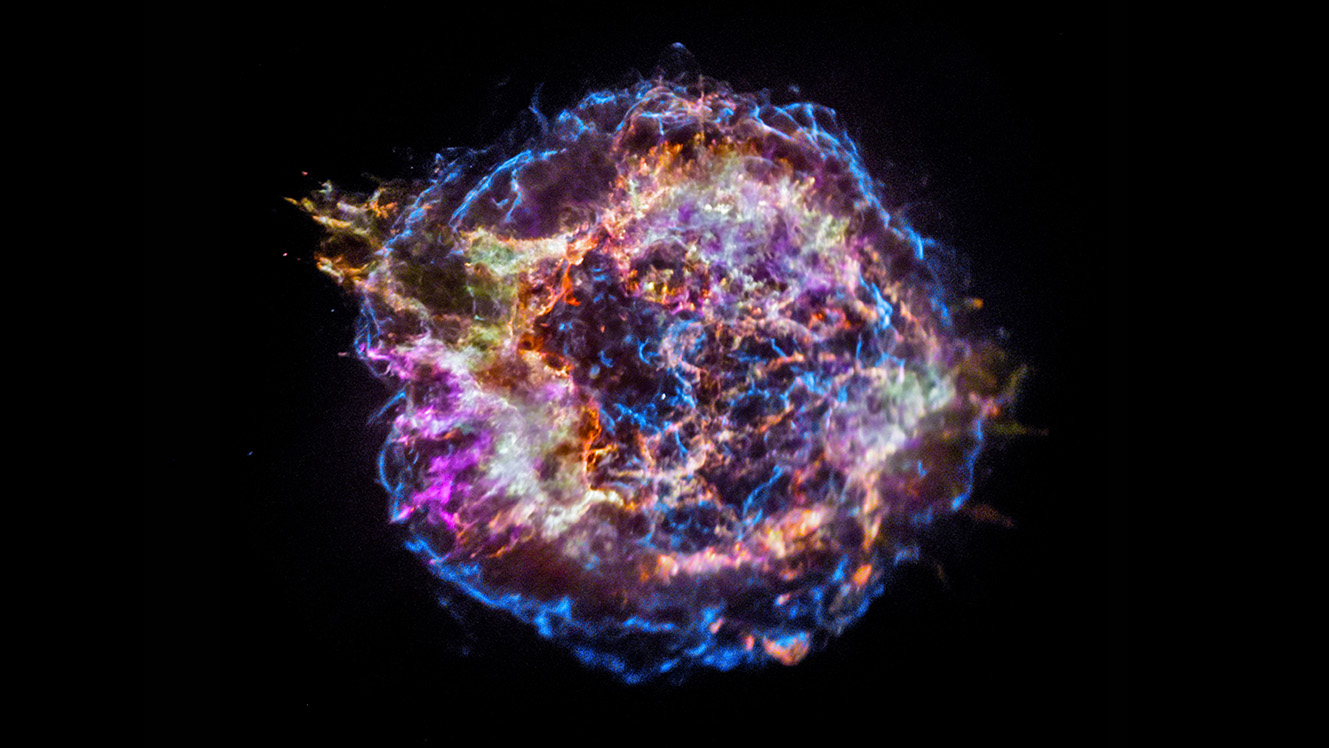
This image of the supernova Cassiopeia A from NASA’s Chandra X-ray Observatory shows the location of different elements in the remains of the explosion: silicon (red), sulfur (yellow), calcium (green) and iron (purple). Each of these elements produces X-rays within narrow energy ranges, allowing maps of their location to be created. Image credit: NASA/CXC/SAO | › Full image and caption
While Chandra has earned its name as one of “The Great Observatories,” astronomers have long desired to peer further into highly energetic environments in space by capturing them in even more detail.
IXPE expands upon Chandra’s work with the introduction of a tool called a polarimeter, an instrument used to understand the shape and direction of the light that reaches the space telescope's detectors. The polarimeter on IXPE allows scientists to gain insight into the finer details of black holes, supernovas, and magnetars, like which direction they are spinning and their three-dimensional shape.

This image of Cassiopeia A was created using some of the first X-ray data collected by IXPE, shown in magenta, combined with high-energy X-ray data from Chandra, in blue. Image credit: NASA/CXC/SAO/IXPE | › Full image and caption
While scientists have just begun putting IXPE's capabilities to use, they're already starting to reveal new details about the inner workings of these objects – such as the magnetic field environment around Cas A, shown in a newly released image.
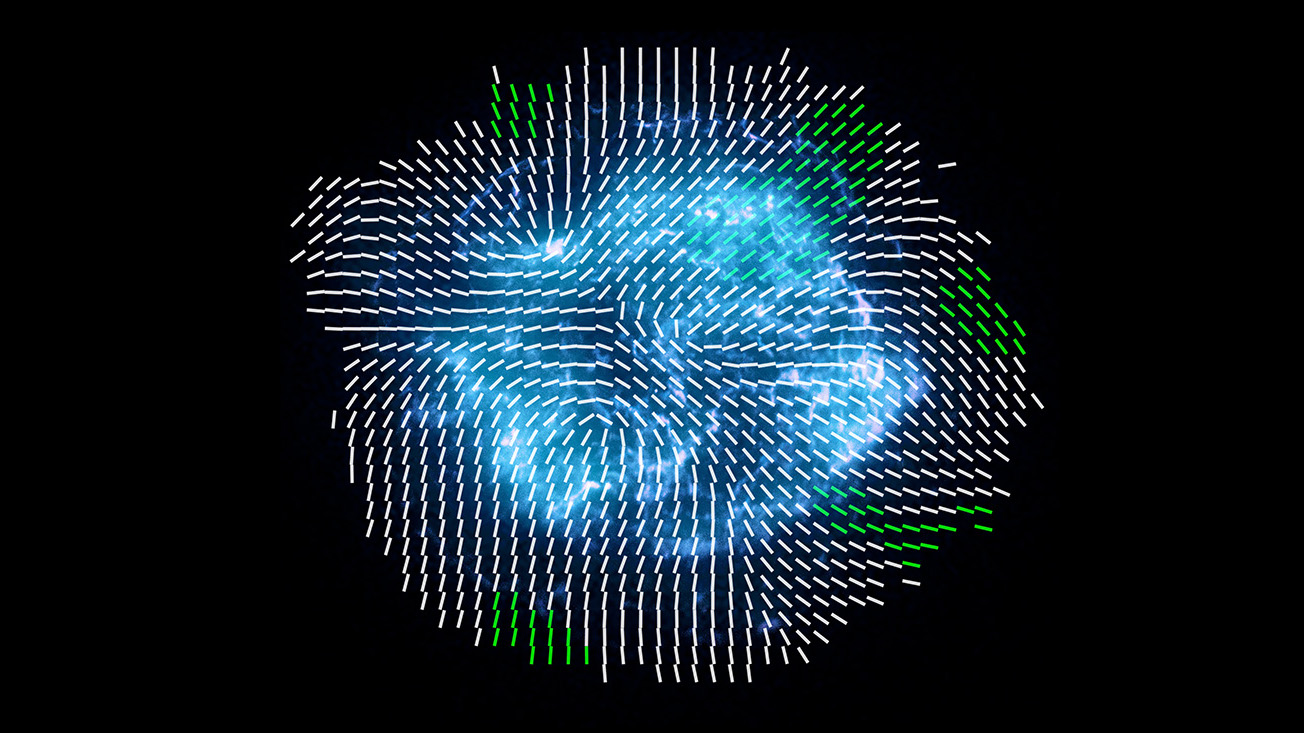
The lines in this newly released image come from IXPE measurements that show the direction of the magnetic field across regions of Cassiopeia A. Green lines indicate regions where the measurements are most highly significant. These results indicate that the magnetic field lines near the outskirts of the supernova remnant are largely oriented radially, i.e., in a direction from the center of the remnant outwards. The IXPE observations also reveal that the magnetic field over small regions is highly tangled, without a dominant preferred direction. Observations such as this one can help scientists learn how particles shooting out from supernovae interact with the magnetic field created by the explosion. Image credits: X-ray: Chandra: NASA/CXC/SAO; IXPE: NASA/MSFC/J. Vink et al. | + Expand image | › Full image and caption
“For the first time, we will use every collected photon of light to tell us about the nature and shapes of objects in the sky that would be dots of light otherwise,” says Roger Romani, a Stanford professor and the co-investigator on IXPE.
How It Works
Generally, when light is produced, it is what we call unpolarized, meaning that it oscillates in every direction. For example, our Sun produces unpolarized light. But sometimes, light is produced in a highly organized fashion, oscillating only in one direction. In astronomy, this arises when magnetic fields force particles to incredibly high speeds, creating highly organized, or polarized, light.
This is what makes objects like the supernova Cas A such enticing targets for IXPE. Exploded stars like Cas A generate massive energetic waves when they go supernova, giving scientists a view of how particles shooting out at immense speeds interact with the magnetic fields from such an event. In the case of Cas A, IXPE was able to determine that the x-rays are not very polarized, meaning the explosion created very turbulent regions with multiple field directions.
While the idea of polarized or organized light may sound abstract, you may have noticed it the last time you were outside on a sunny day. If you’ve tried on a pair of polarized sunglasses, you may have noticed that the glare was greatly reduced. That’s because as light scatters, it bounces off of reflective surfaces in all directions. However, polarized lenses have tiny filters that only allow light coming from a narrow band of directions to pass through.
The polarimeter on IXPE works in a similar way. Astronomers can determine the strength of an object's magnetic field by using the polarimeter to measure how much of the light detected by the telescope is polarized. Typically, the more polarized the light the stronger the magnetic field at the source.
Astronomers can even go a step further to measure the direction this light is oscillating by measuring the angle of the light that reaches the telescope. Because the polarized light leaves the source in a predictable fashion – namely perpendicular from its magnetic field – knowing the angle of the oscillating light provides information about the axis of rotation and potentially even the surface structure of objects such as neutron stars and nebulae.

In this demonstration, the rope represents light waves and the open window represents a polarimeter. Depending on the angle of the light waves (rope), more or less information makes it through the polarimeter (window) the narrower it is. By measuring the amount of light received through the polarimeter, IXPE can determine the angle and the polarization of the light. Image credit: NASA/JPL-Caltech | + Expand image
Imagine, for example, that you were holding one end of a piece of rope secured to an object at the other end. If you swung the rope side to side to make horizontal waves, those waves would be able to make it through a narrow target like a window. If you started to shut the window from the top, narrowing the opening, the waves could conceivably still make it through the opening. However, if you made veritcal waves by waving the rope up and down, as the window closed, fewer and fewer waves would make it through the opening. Likewise, by measuring the light that makes it through the polarimeter to the detector on the other side, IXPE can determine the angle of the light received.
To collect this light, IXPE uses three identical mirrors at the end of a four meter (13 foot) boom. The light received by IXPE is carefully focused on the spacecraft’s polarimeter at the other end of the boom, allowing scientists to collect those crucial measurements.
During the IXPE launch broadcast, commentators discuss the components of the spacecraft and how it measures polarization. | Watch on YouTube
Why It's Important
Building on Chandra's observations from the past two decades, IXPE's novel approach to X-ray science is pulling the curtain back even farther on some of the most fascinating objects in the universe, providing first looks at how and where radiation is being produced in some of the most extreme environments in the universe. IXPE's measurements of Cas A are just the beginning, with even more mysterious targets ready to be explored.
Take it from Martin Weisskopf, the principal scientist on IXPE and project scientist for Chandra, who has spent his 50-year career working in X-ray astronomy, who says, “IXPE will open up the field in ways we’ve been stuck only theorizing about."
Teach It
Explore more on how NASA uses light to map our universe, and dig deeper into some of the celestial features it allows to study, such as blackholes and neutron stars.
Activities
- Project
Exploring Materials - Polarizers
In this activity, learners experiment how polarizers affect light by using two polarizing sheets and overlapping layers of transparent tape.
- Activity Guidebook
Girls STEAM Ahead with NASA Program Cookbook
A guidebook for facilitators planning their own Girls STEAM Ahead with NASA event using NASA’s Universe of Learning resources.
- Slideshow
Black Holes: By the Numbers
What are black holes and how do they form? Explore more in this slideshow.
- Video
What Is a Black Hole?
Find out how what a black hole is, how they can form and why they are so cool!
Educator Resources
-
Science Briefing: Exploring the High Energy Universe
Explore the event materials from this science briefing to get a window into the most energetic processes and the most extreme objects in the universe.
Subject Science
-
The Science of Color
Quickly and easily model how colors reflect, absorb, and interact with each other in the classroom or online using your computer’s camera.
Subject Science
Grades 2-8
Time < 30 mins
-
Using Light to Study Planets
Students build a spectrometer using basic materials as a model for how NASA uses spectroscopy to determine the nature of elements found on Earth and other planets.
Subject Science
Grades 6-11
Time 2+ hrs
-
Dropping In With Gravitational Waves
Students develop a model to represent the collision of two black holes, the gravitational waves that result and the waves' propagation through spacetime.
Subject Science
Grades 6-8
Time 30-60 mins
- Teachable Moments
Telescopes Get Extraordinary View of Milky Way's Black Hole
Find out how scientists captured the first image of Sagittarius A*, why it's important, and how to turn it into a learning opportunity for students.
- Teachable Moments
How Scientists Captured the First Image of a Black Hole
Find out how scientists created a virtual telescope as large as Earth itself to capture the first image of a black hole's silhouette.
- Teachable Moments
Gravitational Waves Detected for the First Time
Find out how researchers proved part of Albert Einstein’s Theory of General Relativity, then create a model of the Nobel Prize-winning experiment in the classroom.
Explore More
- Article: NASA’S IXPE Helps Unlock the Secrets of Famous Exploded Star
- Educator Guide: Black Hole Math
- Opportunity: NASA/IPAC TeacherArchive Research Program
- Student Resources: Chandra
- Article: Hubble - Black Holes
- Interactive: Sagittarius A*
- Video: Hubble - Black Holes
- Website: NASA Science - Black Holes
- Download: A Galaxy Full of Black Holes Presentation
- Expert Talk: Imaging a Black Hole Lecture
- Facts & Figures: NASA - Black Holes
- Article: Black Hole Image Makes History
- Infographic: Anatomy of a Black Hole
NASA's Universe of Learning materials are based upon work supported by NASA under award number NNX16AC65A to the Space Telescope Science Institute, working in partnership with Caltech/IPAC, Center for Astrophysics | Harvard & Smithsonian, and the Jet Propulsion Laboratory.
TAGS: Universe, Stars and Galaxies, Space Telescope, IXPE, Astronomy, Science, Electromagnetic Spectrum, Universe of Learning
Teachable Moments | August 9, 2022
Webb Telescope Sees the Universe Like We've Never Seen It Before
Here's what we learned from the first set of images captured by NASA's newest space observatory and how to translate it into learning opportunities for students.
NASA’s newest space observatory, the James Webb Space Telescope, has returned its first set of images and spectra of five different targets – from nebulae to exoplanets to galaxy clusters – revealing the universe in ways never before seen. These targets, selected by a team of experts, represent just the start of the telescope's science operations and the beginning of our ability to see the universe in a whole new way.
Read on to learn more about what the space-based observatory’s images can tell us about the cosmos, how they were captured, and how to engage learners in the science and engineering behind the mission.
What JWST Saw
New Details Revealed About the Birth of Stars
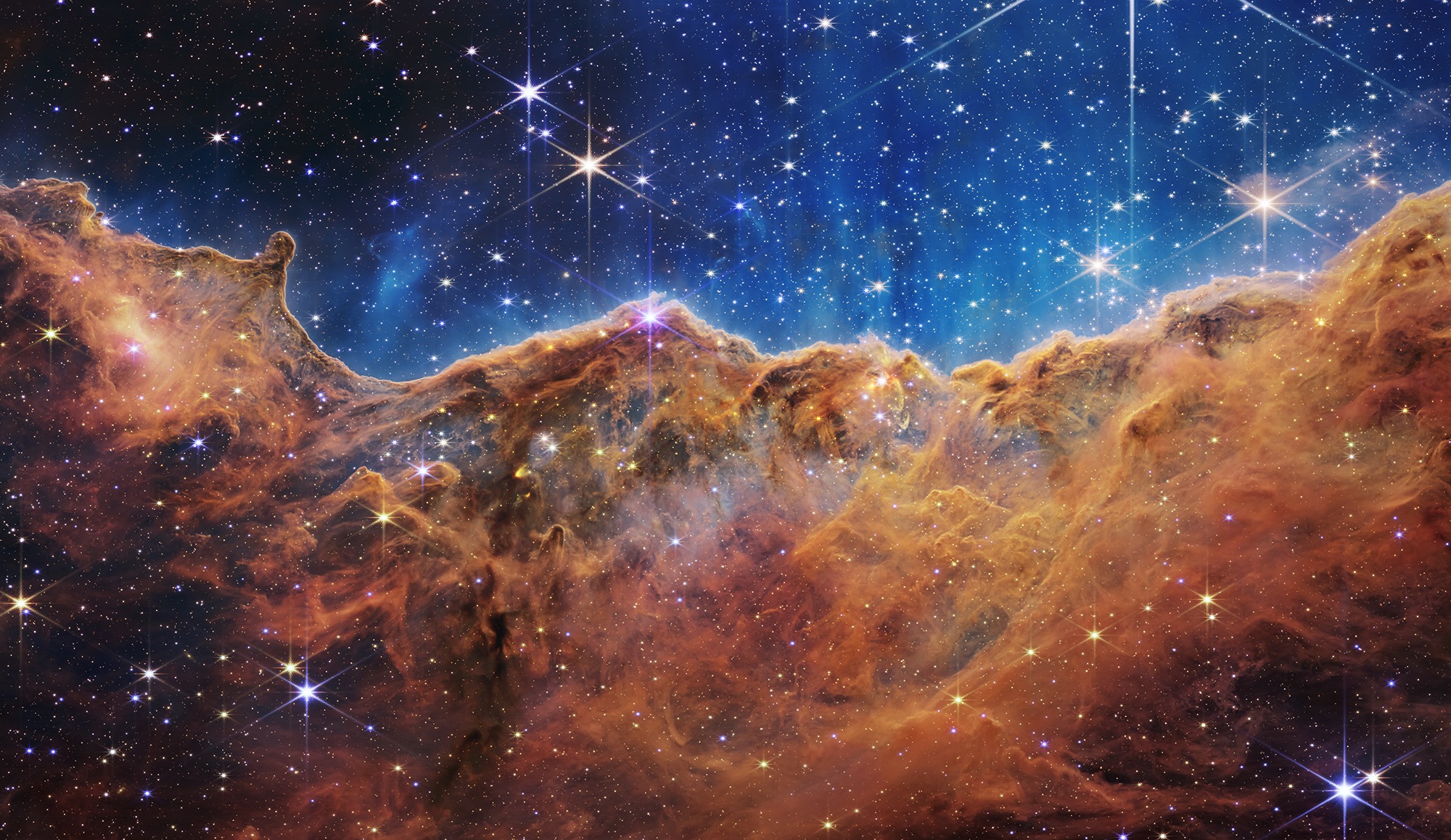
What looks much like craggy mountains on a moonlit evening is actually the edge of a nearby, young, star-forming region NGC 3324 in the Carina Nebula. Captured in infrared light by the Near-Infrared Camera (NIRCam) on NASA’s James Webb Space Telescope, this image reveals previously obscured areas of star birth. Credit: NASA, ESA, CSA, STScI | › Full image and caption | › Text description (PDF)
Stellar nurseries, young stars, and protostellar jets, which are narrow, ultra-fast streams of gas emanating from baby stars, are all on display in this image of the Carina Nebula, a cloud of gas and dust approximately 7,600 light years away.
Nebulae are massive clouds of gas and dust, some spanning up to hundreds of light-years across. Thanks to its infrared cameras, JWST can peer into these dusty regions of space, revealing incredible details previously unseen by other telescopes.
Within the Carina Nebula, a star-forming region known as NGC 3324 was captured by the Webb telescope in this image. As the edge of this region moves inward toward the gas and dust, it may encounter unstable areas. The pressure changes can cause the gas and dust to collapse, forming a new star in a process called accretion. However, if too much material is pushed away, it may prevent a star from forming.
The Webb telescope’s observations in nebulae like this will help scientists answer some of the unknown questions of astrophysics, like what determines the number of stars in a certain region and why do stars form with certain masses.
Scientists can also learn how star formation affects these clouds. Little is known about the numerous small, or low-mass, stars within nebulae. But by studying the jets revealed in the new image, scientists can understand how these stars are expelling gas and dust out of the cloud, thereby reducing the amount of material available to form new stars. Furthermore, scientists will be able to get a full count of these low-mass stars and account for their impact throughout the nebula.
Signs of Water on a Distant Planet

A transmission spectrum made from a single observation using Webb’s Near-Infrared Imager and Slitless Spectrograph (NIRISS) reveals atmospheric characteristics of the hot gas giant exoplanet WASP-96 b. Credits: NASA, ESA, CSA, STScI | › Full image and caption | › Text description (PDF)
JWST's observations of exoplanet WASP-96 b, a planet outside our solar system, is not an image but a spectrum of light. Within the spectrum are highlights that indicate the presence of water molecules. The spectrum also shows evidence of clouds and haze, which were thought not to exist in WASP-96 b's atmosphere.
WASP-96 b is an exoplanet made up mostly of gas. About half the mass of Jupiter, but slightly larger, it orbits much closer to its star, completing a revolution every 3.4 days compared with 12 years for Jupiter.
This measurement, known as a transmission spectrum, was collected as WASP-96 b transited, or passed in front of, its host star from the perspective of the telescope. It compares the light that passed through the atmosphere of the exoplanet with the light from the planet's parent star alone. As a result, it is possible to see the amount of light at each wavelength blocked by the planet and absorbed by its atmosphere, telling scientists the size of the planet and the chemicals contained in its atmosphere.
While WASP-96 b’s spectrum had been captured before, the Webb telescope provided the most detailed view of its spectrum in near-infrared, and the improved resolution completely changed our understanding of the exoplanet’s atmosphere. Using this spectrum, scientists will be able to measure the amount of water in the exoplanet's atmosphere, determine how much oxygen and carbon it contains, infer the make-up of the planet, and even how, when, and where it formed.
Star Pair Coming Into Focus
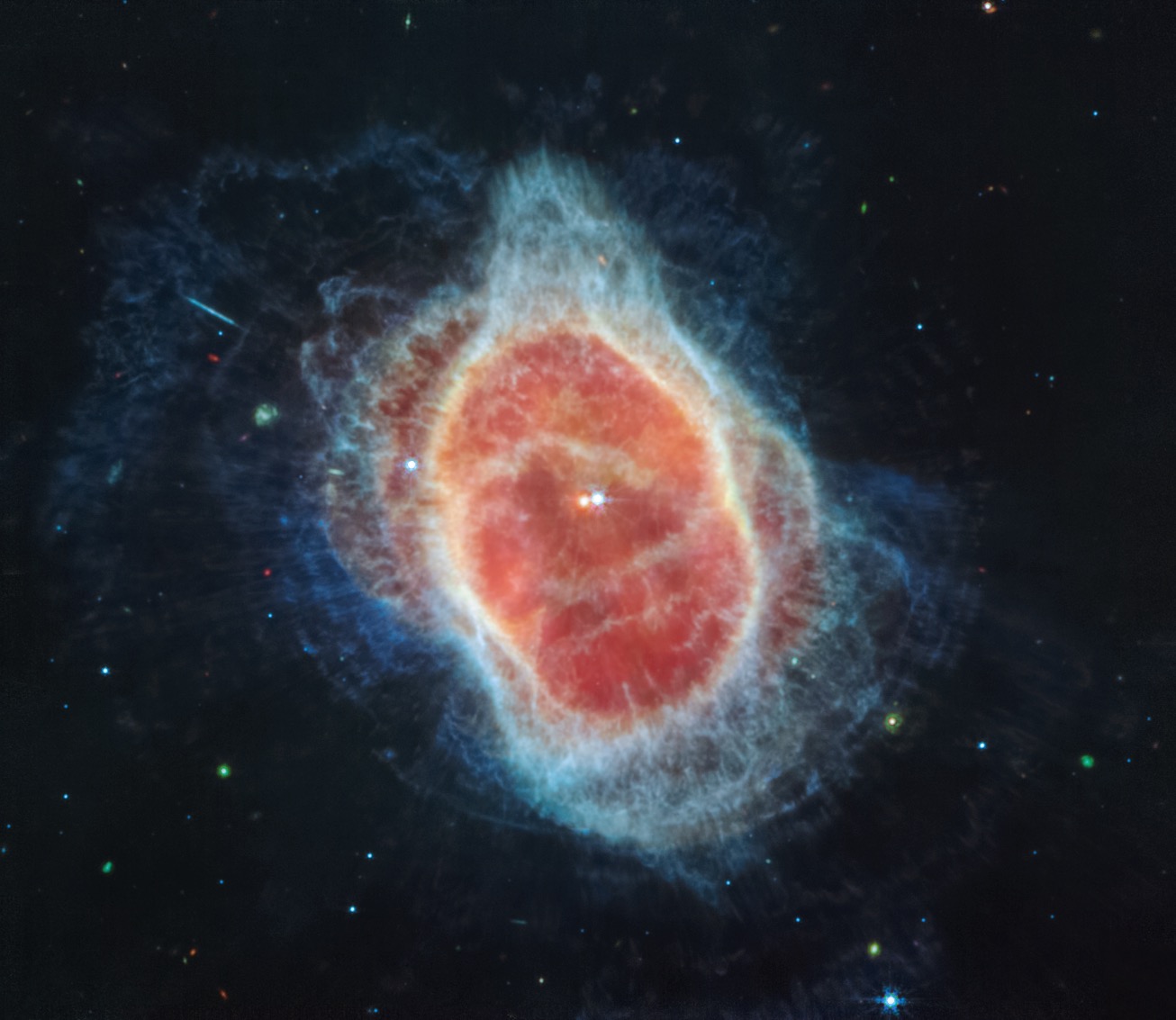
NASA’s Webb Telescope has revealed the cloak of dust around the second star, shown at left in red, at the center of the Southern Ring Nebula for the first time. It is a hot, dense white dwarf star. Credits: NASA, ESA, CSA, STScI | › Full image and caption | › Text description (PDF)
Two distinct stars can be seen in this image of the center of the Southern Ring Nebula – a pairing that was believed to exist but was not visible in previous images.
The star pair came into view thanks to the space telescope's MIRI instrument, which is designed to capture wavelengths of light in the mid-infrared range of the electromagnetic spectrum. MIRI’s ability to see in the mid-infrared revealed that the older of the two stars is surrounded by dust. Seeing this dust clearly is what makes the second star visible in the image. While the brighter star is in an earlier stage of its life, it will likely form its own planetary nebula in the future.
About 2,500 light-years away from Earth, the Southern Ring is a planetary nebula – a shell of gas and dust shed from a dying white dwarf star at its center. Its gases stretch out for nearly half a light-year and are speeding away from the star at approximately nine miles per second!

The bright star at the center of NGC 3132, while prominent when viewed by NASA’s Webb Telescope in near-infrared light, plays a supporting role in sculpting the surrounding nebula. A second star, barely visible at lower left along one of the bright star’s diffraction spikes, is the nebula’s source. It has ejected at least eight layers of gas and dust over thousands of years. Credits: NASA, ESA, CSA, STScI | › Full image and caption | › Text description (PDF)
The images from JWST reveal that starlight streams out of the nebula in fine lines, the result of holes in the surrounding gas and dust cloud. The types and locations of different molecules within the cloud, gleaned from the captured spectra, will help to fine tune our understanding of the structure, composition, and history of this nebula, and with future observations, other nebulae.
How Galaxies Interact

An enormous mosaic of Stephan’s Quintet is the largest image to date from NASA’s James Webb Space Telescope, covering about one-fifth of the Moon’s diameter. It contains over 150 million pixels and is constructed from almost 1,000 separate image files. The visual grouping of five galaxies was captured by Webb’s Near-Infrared Camera (NIRCam) and Mid-Infrared Instrument (MIRI). Credits: NASA, ESA, CSA, STScI | › Full image and caption | › Text description (PDF)
The Webb telescope's capabilities bring new eyes to a cluster of galaxies first discovered in 1877 and known as Stephan’s Quintet. On display in this sharp new image are regions of new star birth containing millions of young stars as well as tails of gas, dust, and stars being ripped from galaxies as a result of gravitational forces between the galaxies.
Stephan’s Quintet is a dense cluster of galaxies located 290 million light-years away in the constellation Pegasus. Four of the five galaxies within the quintet are locked in orbits that repeatedly bring them close to one another. The fifth (leftmost) galaxy is seven times closer to Earth than the others. But its location within the line of sight of the distant four makes it appear to be grouped with them. What looks like speckles surrounding the nearby galaxy and could be mistaken for digital noise is actually individual stars from that galaxy.
It may seem distant in pure numbers, but Stephan's Quintet is relatively close compared to galaxies that are billions of light-years away. Its proximity gives astronomers a great view of the interactions that occur between galaxies that are near to each other.
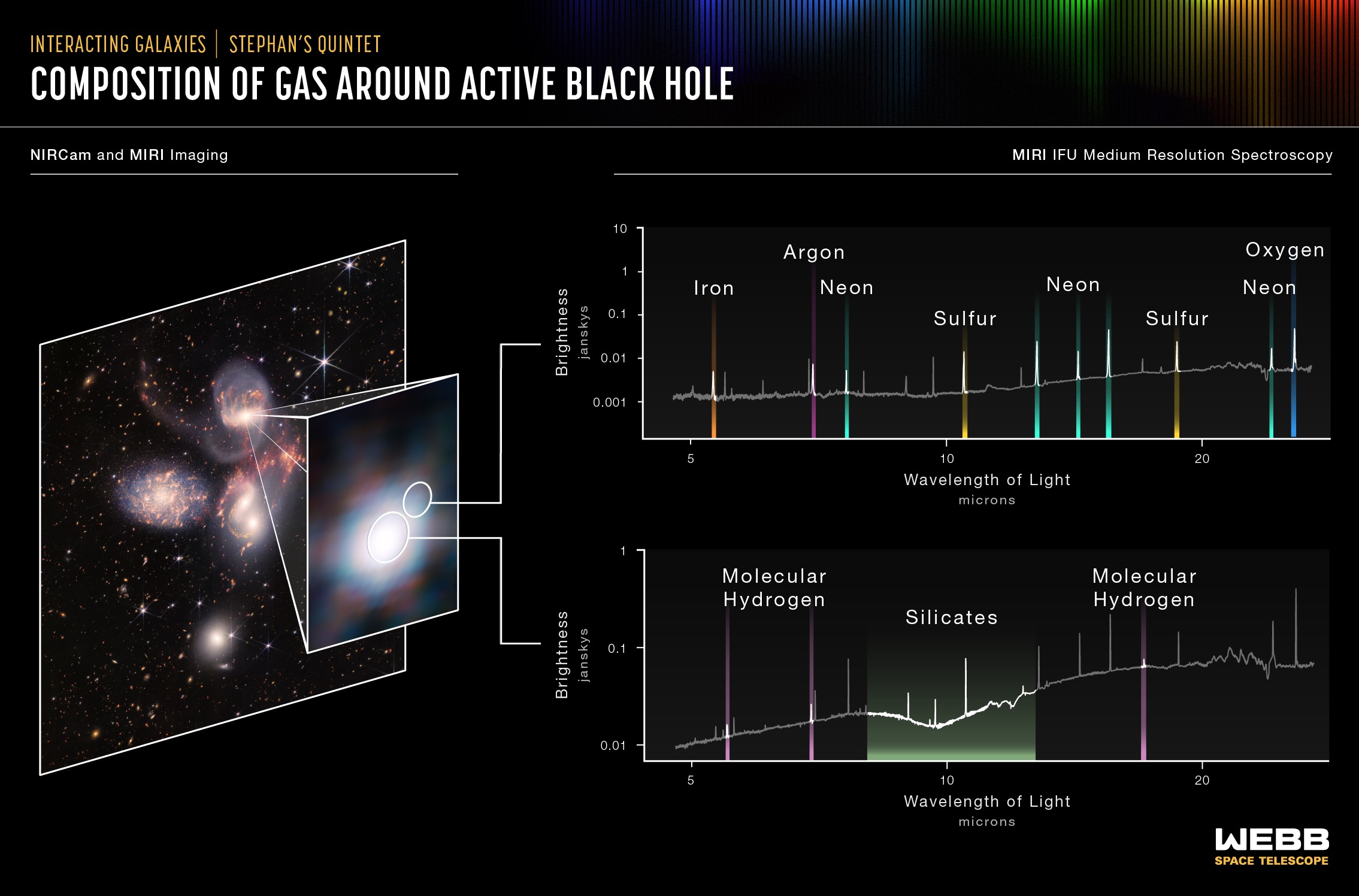
The MIRI instrument measured the composition of gas around the black hole at the center of one of the galaxies in Stephan's Quintent to find a region filled with hot, ionized gases, including iron, argon, neon, sulfur and oxygen (top spectrum). The instrument also revealed that the supermassive black hole has a reservoir of colder, denser gas with large quantities of molecular hydrogen and silicate dust (bottom spectrum). Credits: NASA, ESA, CSA, STScI | › Full image and caption | › Text description (PDF)
The detail exposed will allow scientists to understand the interactions occurring in much more distant – and harder to observe – galaxies. Close inspections of galactic nuclei captured in mid-infrared by the telescope's MIRI instrument revealed hot gas being stripped of its electrons by winds and radiation from a supermassive black hole at the center of one galaxy. The new detail helped scientists determine that iron, argon, neon, sulfur and oxygen, as well as silicate dust are contained in these gases.
Meanwhile, the telescope's NIRSpec instrument – which can capture up to 100 spectra at a time – was able to identify atomic and molecular hydrogen as well as iron ions in the gases around the black hole. These observations will provide a greater understanding about the rate at which supermassive black holes feed and grow.
Thousands of Galaxies in a Grain of Sand
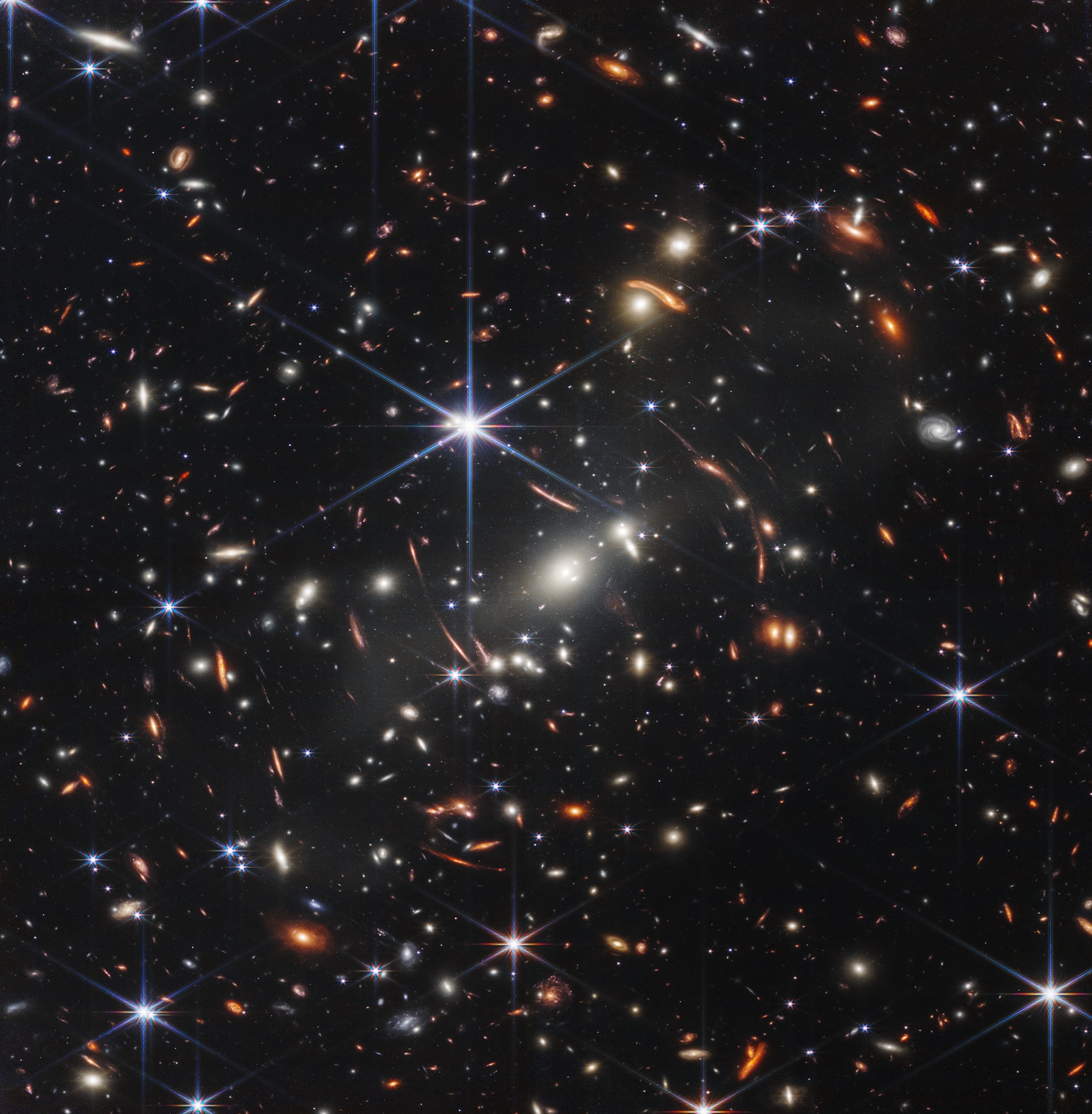
NASA’s James Webb Space Telescope has produced the deepest and sharpest infrared image of the distant universe to date. Known as Webb’s First Deep Field, this image of galaxy cluster SMACS 0723 is overflowing with detail. Credits: NASA, ESA, CSA, STScI | › Full image and caption | › Text description (PDF)
This image contains thousands of galaxies as well as the faintest objects yet observed in the infrared. Known as a deep field image, it was made by pointing the telescope at the target for an extended period of time, allowing the detectors to collect as much of the faint, distant light as possible. JWST captured this deep field image in just 12.5 hours, while the Hubble Space Telescope spent two weeks capturing its deepest images. (Note that Hubble also observed this galaxy cluster, but not as a deep field image.)
Hold a single grain of sand at arm's length and you could cover the entire area of space captured by this image. Keen observers will notice what appear as warped or stretched galaxies. Those are the result of gravitational lensing, a phenomenon in which the gravity of the galaxy cluster centered in the foreground bends the light from background galaxies magnifying and distorting their light. Taking advantage of this phenomenon allows for viewing of extremely distant and very faint galaxies.
The galaxy cluster shown in the image is known as SMACS 0723 and it appears as it did 4.6 billion years ago – the length of time it took for its light to reach the telescope. Light from the oldest-known galaxy in the image had been traveling for 13.1 billion years before it reached JWST.
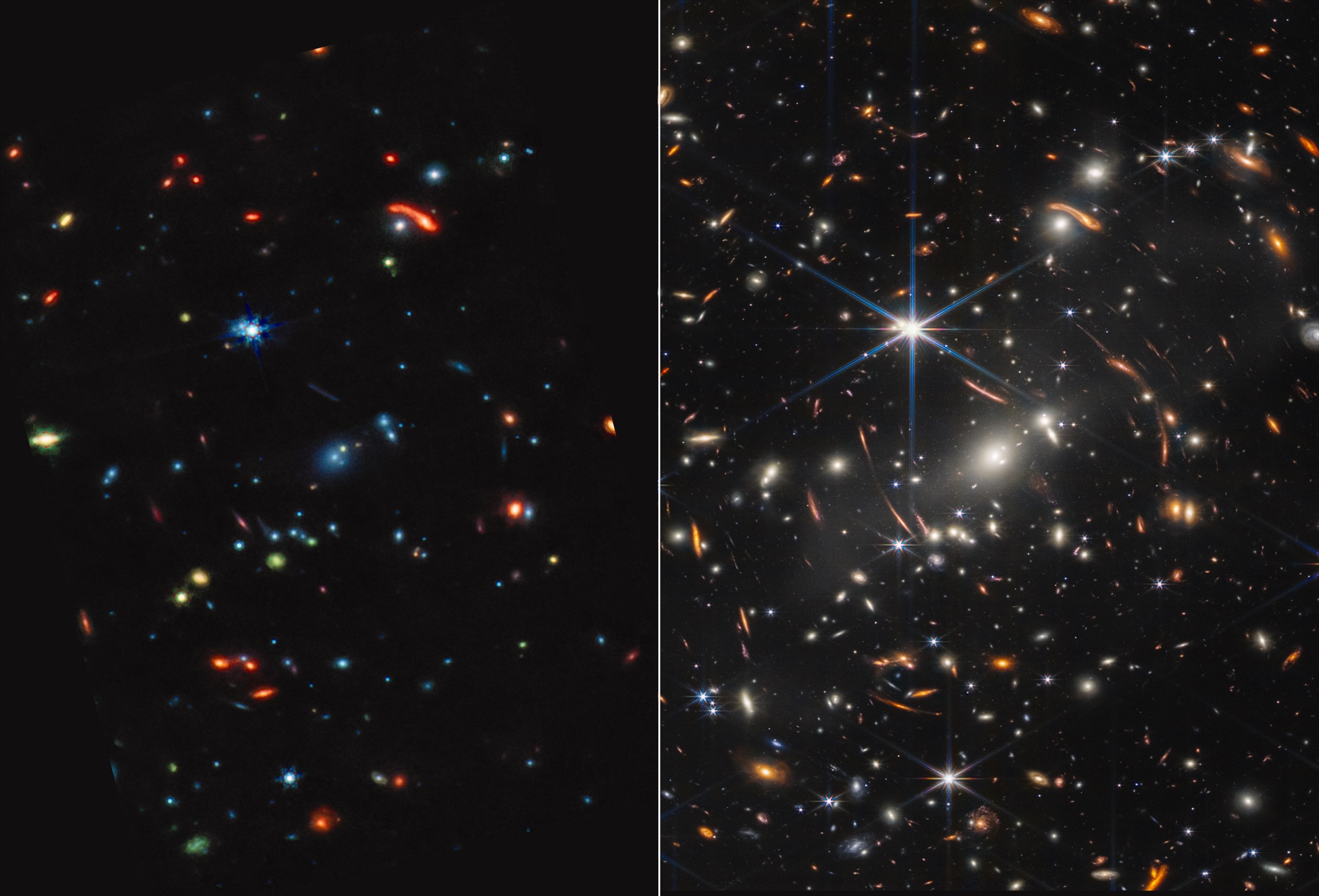
Galaxy cluster SMACS 0723 is a technicolor landscape when viewed in mid-infrared light by NASA’s James Webb Space Telescope. Compared to Webb’s near-infrared image at right, the galaxies and stars are awash in new colors. Credits: NASA, ESA, CSA, STScI | › Full image and caption | › Text description (PDF)
The MIRI instrument’s ability to detect longer infrared wavelengths provides additional information in the image about the make-up of those galaxies. Mid-infrared light highlights dust, an important star-forming ingredient. In this image, red objects contain thick dust layers, while blue galaxies contain stars but not much dust. Green objects contain dust filled with hydrocarbons and other compounds. With these data, astronomers will be able to better understand the formation and growth of galaxies.
As impressive as this image is, the JWST team has plans to capture more deep field images using even longer exposure times. Keep up to date with the latest images and spectra from JWST throughout the school year at the Webb Space Telescope Resource Gallery.
How They Did It
The Webb telescope's ability to detect these objects in such great detail is enabled by its size, the way it observes the universe, and the unique technologies aboard. We went into more detail about how JWST works in a previous Teachable Moment, but below you’ll find a review of some of the important ways JWST was uniquely designed to capture these groundbreaking images.
Observing the Infrared

Light waves get stretched as the universe expands similar to how this ink mark stretches out as the elastic is pulled. Get students modeling and exploring this effect with this standards-aligned math lesson. Credit: NASA/JPL-Caltech | + Expand image
The Webb telescope was built with instruments like NIRSpec and MIRI that are sensitive to light in the near- and mid-infrared wavelengths to detect some of the most distant objects in space.
As light from distant objects travels to Earth, the universe expands, something it’s been doing since the Big Bang. The waves that make up the light get stretched as the universe expands. Visible lightwaves from distant objects that you would be able to see with your eyes get stretched out so far that the longer wavelengths shift from visible light into infrared. Scientists refer to this phenomenon as redshift – and the farther away an object is, the more redshift it undergoes.
Gathering Light
The light from some of these distant objects has traveled so far that it is incredibly dim. To see such dim light, the Webb telescope was built to be extremely sensitive. On the Webb telescope, 18 hexagonal mirrors combine to form a massive primary mirror that is 21 feet (6.5 meters) across – six times the surface area of Hubble.
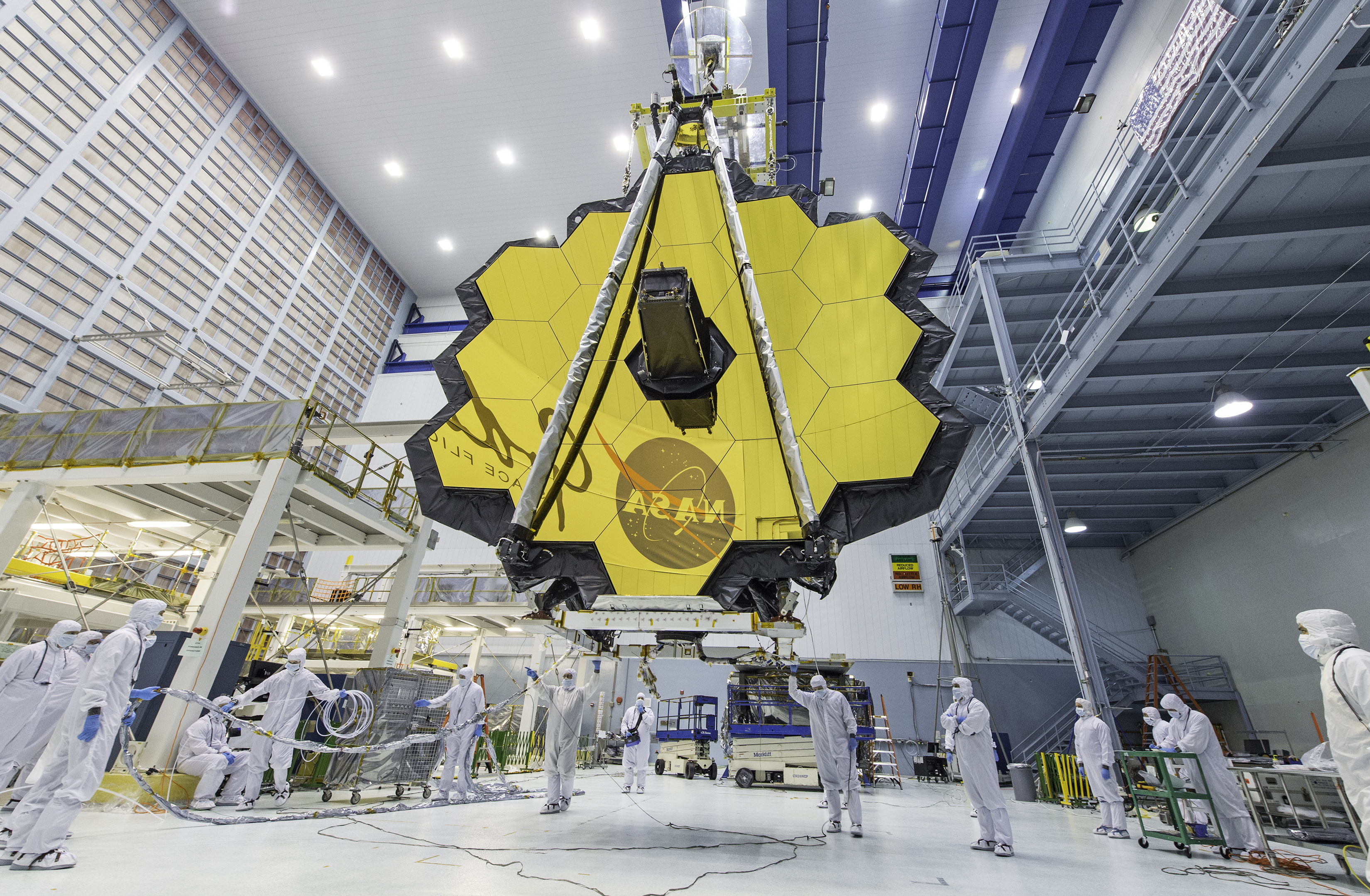
The complete optical telescope element on display inside a clean room at NASA’s Goddard Space Flight Center in Greenbelt, Maryland, in 2017. Credits: NASA/Desiree Stover | › Full image and caption
To detect faint infrared light, the instruments inside the telescope have to be kept very cold, otherwise those infrared signals could get lost in the heat of the telescope. The spacecraft’s orbit and tennis-court-size sunshield keep light and heat from the Sun, Earth, and Moon from warming up its sensitive instruments. And the MIRI instrument, which needs to be even colder to capture mid-infrared wavelengths, is equipped with a special cryocooler.
The unique design and innovative techniques used by the James Webb Space Telescope are what made the first images possible. As the mission continues, more targets will be observed, more discoveries will be made, and more of our universe will unfold before our eyes.
“It’s not every day you can say you contributed to something that inspires the world in a positive way, but I believe that’s what JWST is doing for everyone of all ages,” said JPL engineer Analyn Schneider, who is the project manager for the telescope's MIRI instrument. “The telescope will help us learn more about our galaxy and the rest of the universe, and as a bonus we get these magnificent images. Learning is a big part of being in science and engineering and that’s what makes it interesting and challenging.”
Teach It
Bring the excitement of these far-off observations closer to home by using the following resources in your learning environment, whether in-person, hybrid, or remote. Scientists and educators directly connected with the James Webb Space Telescope have teamed up to provide a collection of Webb resources to meet your needs. Find additional resources below and through NASA’s Universe of Learning project.
Lessons
-
The Expanded Universe: Playing With Time Activity Guide
In this activity, participants use balloons to model the expansion of the universe and observe how expansion affects wavelengths of light and distance between galaxies.
-
Modeling the Orbits of Planets
Students use a planar model of a gravity well to create different orbital configurations around a central mass.
Subject Science
Grades 6-12
Time 30-60 mins
-
Math of the Expanding Universe
Students will learn about the expanding universe and the redshift of lightwaves, then perform their own calculations with a distant supernova.
Subject Science
Grades 9-12
Time 30-60 mins
-
The Science of Color
Quickly and easily model how colors reflect, absorb, and interact with each other in the classroom or online using your computer’s camera.
Subject Science
Grades 2-8
Time < 30 mins
-
Calculating Solar Power in Space
Students explore practical applications of exponents and division to investigate what it takes for NASA spacecraft to travel deep into the solar system using only solar power.
Subject Math
Grades 6-8
Time 30-60 mins
-
Using Light to Study Planets
Students build a spectrometer using basic materials as a model for how NASA uses spectroscopy to determine the nature of elements found on Earth and other planets.
Subject Science
Grades 6-11
Time > 2 hrs
-
Collecting Light: Inverse Square Law Demo
In this activity, students learn how light and energy are spread throughout space. The rate of change can be expressed mathematically, demonstrating why spacecraft like NASA’s Juno need so many solar panels.
Subject Math
Grades 6-12
Time < 30 mins
-
Build a Light Detector Inspired by Space Communications
In this advanced programming activity, students will construct a light-wavelength detector to model future technology for communicating with spacecraft.
Subject Technology
Grades 9-12
Time > 2 hrs
-
James Webb Space Telescope STEM Toolkit
Find a collection of resources, activities, videos, and more for your students to learn about NASA’s newest space observatory.
Student Activities
Explore More
-
Planet Pinpointer: A 'Pi in the Sky' Math Challenge
In this illustrated math problem, students use pi to calculate the distance across a disk of debris around the star Beta Pictoris.
Subject Math
Grades 10-12
Time < 30 mins
- Teachable Moments
How Scientists Captured the First Image of a Black Hole
Find out how scientists created a virtual telescope as large as Earth itself to capture the first image of a black hole's silhouette.
- Expert Talk
Teaching Space With NASA – Revealing the Universe With Infrared
In this educational talk, NASA experts discuss how we use non-visible light to explore the universe.
-
Black Holes: By the Numbers
What are black holes and how do they form? Explore more in this slideshow for students.
Subject Science
Grades 4-12
Time < 30 mins
- Slide Deck: James Webb Space Telescope
- Sonification: Exoplanet WASP-96b Spectra
- Interactive: The James Webb Space Telescope Virtual Experience
- Gallery: Cool Cosmos Infrared Galaxy
- Interactive: Viewspace – Star Formation: Eagle Nebula
- Article for Kids: What is a Transit?
- Article for Kids: What is a Light Year?
- Article for Kids: What is a Nebula?
- Article for Kids: What is a Black Hole?
- Article for Kids: What is a Galaxy?
- Article for Kids: Explore the Electromagnetic Spectrum
- Article for Kids: How Old are Galaxies?
- Website: James Webb Space Telescope
- Image Gallery: James Webb Space Telescope Images
- Website: NASA Exoplanets
- Interactive: Eyes on Exoplanets
- Facts & Figures: Exoplanet Catalog
- Downloads: James Webb Space Telescope Poster
- Downloads: Exoplanet Travel Bureau Posters
- Webb Telescope Videos
- Webb Telescope Paper Model
NASA's Universe of Learning materials are based upon work supported by NASA under award number NNX16AC65A to the Space Telescope Science Institute, working in partnership with Caltech/IPAC, Center for Astrophysics | Harvard & Smithsonian, and the Jet Propulsion Laboratory.
TAGS: Stars & Galaxies, JWST, K-12 Education, Teaching, Universe of Learning
Teachable Moments | September 30, 2021
Learn About the Universe With the James Webb Space Telescope
Get a look into the science and engineering behind the largest and most powerful space telescope ever built while exploring ways to engage learners in the mission.
NASA is launching the largest, most powerful space telescope ever. The James Webb Space Telescope will look back at some of the earliest stages of the universe, gather views of early star and galaxy formation, and provide insights into the formation of planetary systems, including our own solar system.
Read on to learn more about what the space-based observatory will do, how it works, and how to engage learners in the science and engineering behind the mission.
What It Will Do
The James Webb Space Telescope, or JWST, was developed through a partnership between NASA and the European and Canadian space agencies. It will build upon and extend the discoveries made by the Hubble Space Telescope to help unravel mysteries of the universe. First, let's delve into what scientists hope to learn with the Webb telescope.
A look at the James Webb Space Telescope, its mission and the incredible technological challenge this mission presents. | Watch on YouTube
How Galaxies Evolve
What the first galaxies looked like and when they formed is not known, and the Webb telescope is designed to help scientists learn more about that early period of the universe. To better understand what the Webb telescope will study, it’s helpful to know what happened in the early universe, before the first stars formed.
The universe, time, and space all began about 13.8 billion years ago with the Big Bang. For the first few hundred-thousand years, the universe was a hot, dense flood of protons, electrons, and neutrons, the tiny particles that make up atoms. As the universe cooled, protons and neutrons combined into ionized hydrogen and helium, which had a positive charge, and eventually attracted all those negatively charged electrons. This process, known as recombination, occurred about 240,000 to 300,000 years after the Big Bang.

This image shows the temperature fluctuations (shown as color differences) in the cosmic microwave background from a time when the universe was less than 400,000 years old. The image was captured by the Wilkinson Microwave Anisotropy Probe, or WMAP, which spent nine years, from 2001 to 2010, collecting data on the early universe. Credit: NASA | › Full image and caption | + Expand image
Light that previously couldn’t travel without being scattered by the dense ionized plasma of early particles could now travel freely. The very first form of light we can look back and see comes from this time and is known as the cosmic microwave background radiation. It is essentially a map of temperature fluctuations across the universe left behind from the Big Bang. The fluxuations give clues about the origin of galaxies and the large-scale structure of galaxies. There were still no stars in the universe at this time, so the next several hundred million years are known as the cosmic dark ages.
Current theory predicts that the earliest stars were big – 30 to 300 times the size of our Sun – and burned quickly, ending in supernova explosions after just a few million years. (For comparison, our Sun has a lifespan of about 10 billion years and will not go supernova.) Observing these luminous supernovae is one of the few ways scientists could study the earliest stars. That is vital to understanding the formation of objects such as the first galaxies.
By using the Webb telescope to compare the earliest galaxies with those of today, scientists hope to understand how they form, what gives them their shape, how chemical elements are distributed across galaxies, how central black holes influence their galaxies, and what happens when galaxies collide.
Learn how the James Webb Space Telescope's ability to look farther into space than ever before will bring newborn galaxies into view. | Watch on YouTube
How Stars and Planetary Systems Form
Stars and their planetary systems form within massive clouds of dust and gas. It's impossible to see into these clouds with visible light, so the Webb telescope is equipped with science instruments that use infrared light to peer into the hearts of stellar nurseries. When viewing these nurseries in the mid-infrared – as the Webb telescope is designed to do – the dust outside the dense star forming regions glows and can be studied directly. This will allow astronomers to observe the details of how stars are born and investigate why most stars form in groups as well as how planetary systems begin and evolve.

This mosaic image is the sharpest wide-angle view ever obtained of the starburst galaxy, Messier 82 (M82). The galaxy is remarkable for its bright blue disk, webs of shredded clouds and fiery-looking plumes of glowing hydrogen blasting out of its central regions.Throughout the galaxy's center, young stars are being born 10 times faster than they are inside our entire Milky Way Galaxy. Credit: NASA, ESA, and The Hubble Heritage Team (STScI/AURA); Acknowledgment: J. Gallagher (University of Wisconsin), M. Mountain (STScI), and P. Puxley (National Science Foundation) | › Full image and caption | + Expand image
How Exoplanets and Our Solar System Evolve
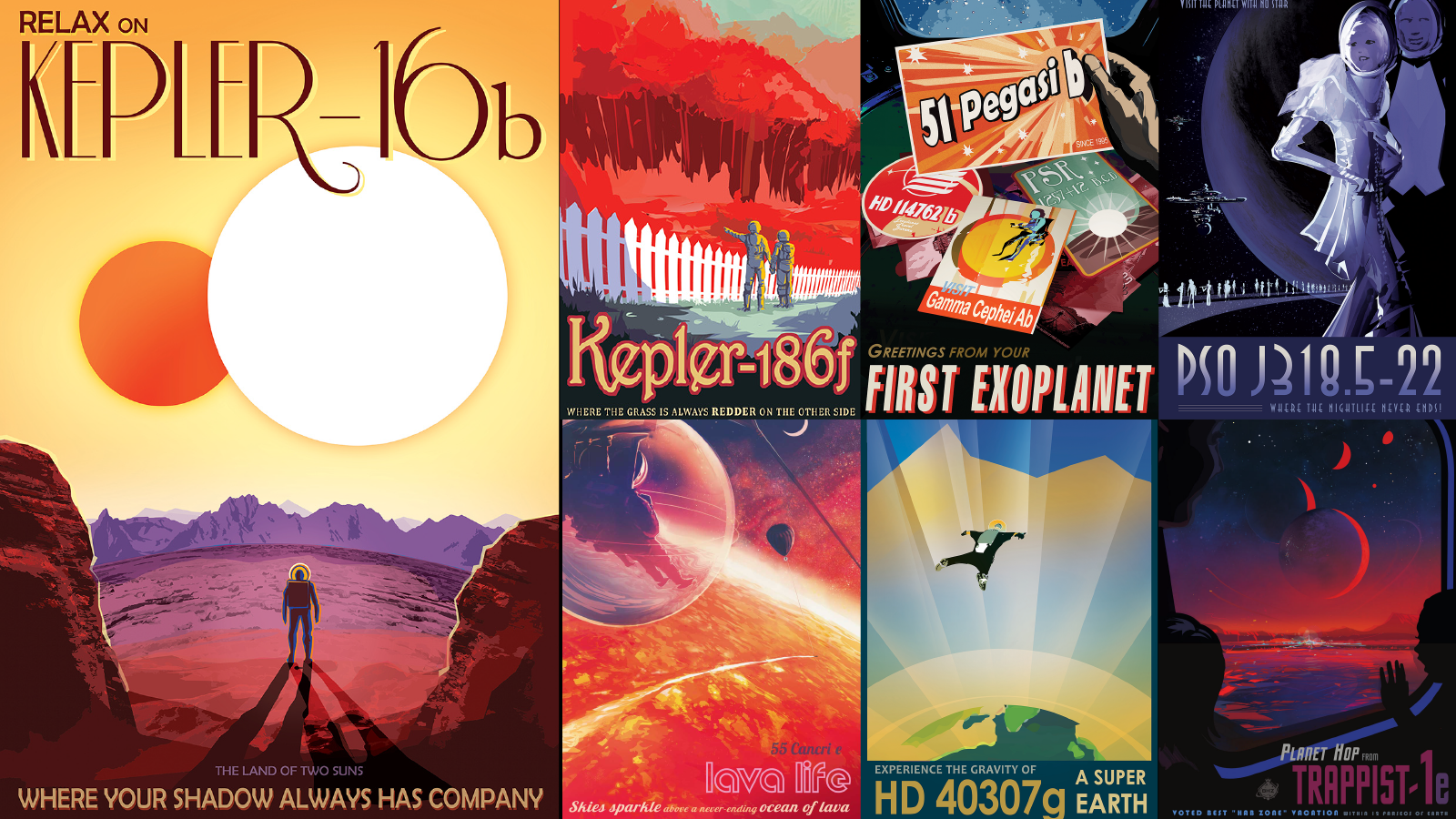
As we make more discoveries about exoplanets, artists at NASA are imagining what future explorers might encounter on these faraway worlds as part of the Exoplanet Travel Bureau poster series. Credit: NASA | › View and download the posters | + Expand image
The first planet outside our solar system, or exoplanet, was discovered in 1992. Since then, scientists have found thousands more exoplanets and estimate that there are hundreds of billions in the Milky Way galaxy alone. There are many waiting to be discovered and there is more to learn about the exoplanets themselves, such as what makes up their atmospheres and what their weather and seasons may be like. The Webb telescope will help scientists do just that.
In our own solar system, the Webb telescope will study planets and other objects to help us learn more about our solar neighborhood. It will be able to complement studies of Mars being carried out by orbiters, landers, and rovers by searching for molecules that may be signs of past or present life. It is powerful enough to identify and characterize icy comets in the far reaches of our solar system. And it can be used to study places like Saturn, Uranus, and Neptune while there are no active missions at those planets.
How It Works
The Webb telescope has unique capabilities enabled by the way it views the universe, its size, and the new technologies aboard. Here's how it works.
Peering Into the Infrared
To see ancient, distant galaxies, the Webb telescope was built with instruments sensitive to light in the near- and mid-infrared wavelengths.
Light leaving these galaxies can take billions of years to reach Earth, so when we see these objects, we’re actually seeing what they looked like in the past. The farther something is from Earth, the farther back in time it is when we observe it. So when we look at light that left objects 13.5 billion years ago, we're seeing what happened in the early universe.
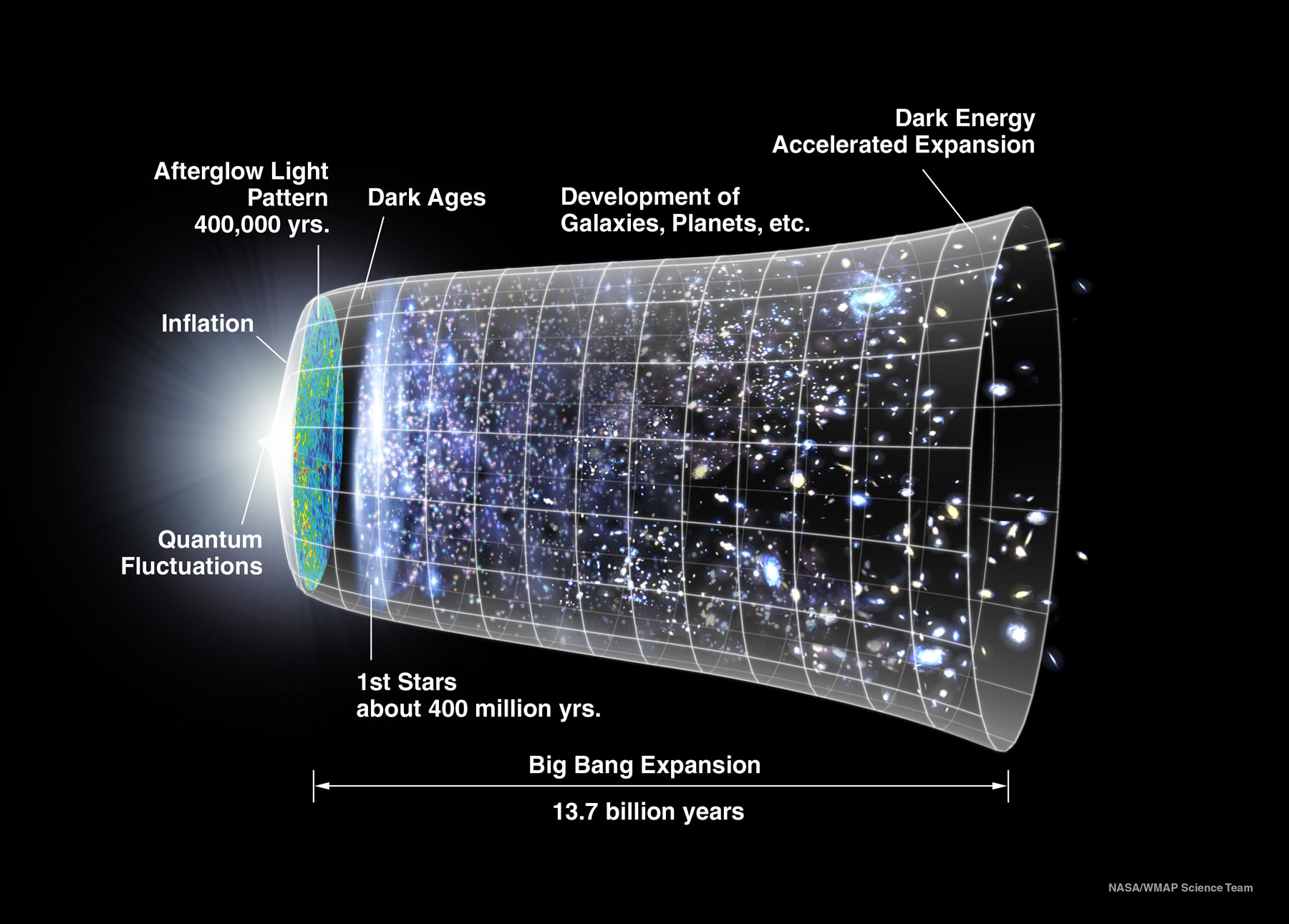
An illustrated timeline of the universe. Credit: WMAP | + Expand image
As light from distant objects travels to Earth, the universe continues to expand, something it’s been doing since the Big Bang. The waves that make up the light get stretched as the universe expands. You can see this effect in action by making an ink mark on a rubber band and observing how the mark stretches out when you pull on the rubber band.

Light waves get stretched as the universe expands similar to how this ink mark stretches out as the elastic is pulled. Get students modeling and exploring this effect with this standards-aligned math lesson. Credit: NASA/JPL-Caltech | + Expand image
What this means for light coming from distant galaxies is that the visible lightwaves you would be able to see with your eyes get stretched out so far that the longer wavelengths shift from visible light into infrared. Scientists refer to this phenomenon as redshift – and the farther away an object is, the more redshift it undergoes.
Webb telescope’s infrared sensing equipment will give scientists the chance to study some of the earliest stars that exploded in supernova events, creating the elements necessary to build planets and form life.
Gathering Light
The first stars were massive, their life cycles ending in supernova explosions. The light from these explosions has traveled so far that it is incredibly dim. This is due to the inverse square law. You experience this effect when a room appears to get darker as you move away from a light source.
To see such dim light, the Webb telescope needs to be extremely sensitive. A telescope’s sensitivity, or its ability to detect faint signals, is related to the size of the mirror it uses to gather light. On the Webb telescope, 18 hexagonal mirrors combine to form a massive primary mirror that is 21 feet (6.5 meters) across.
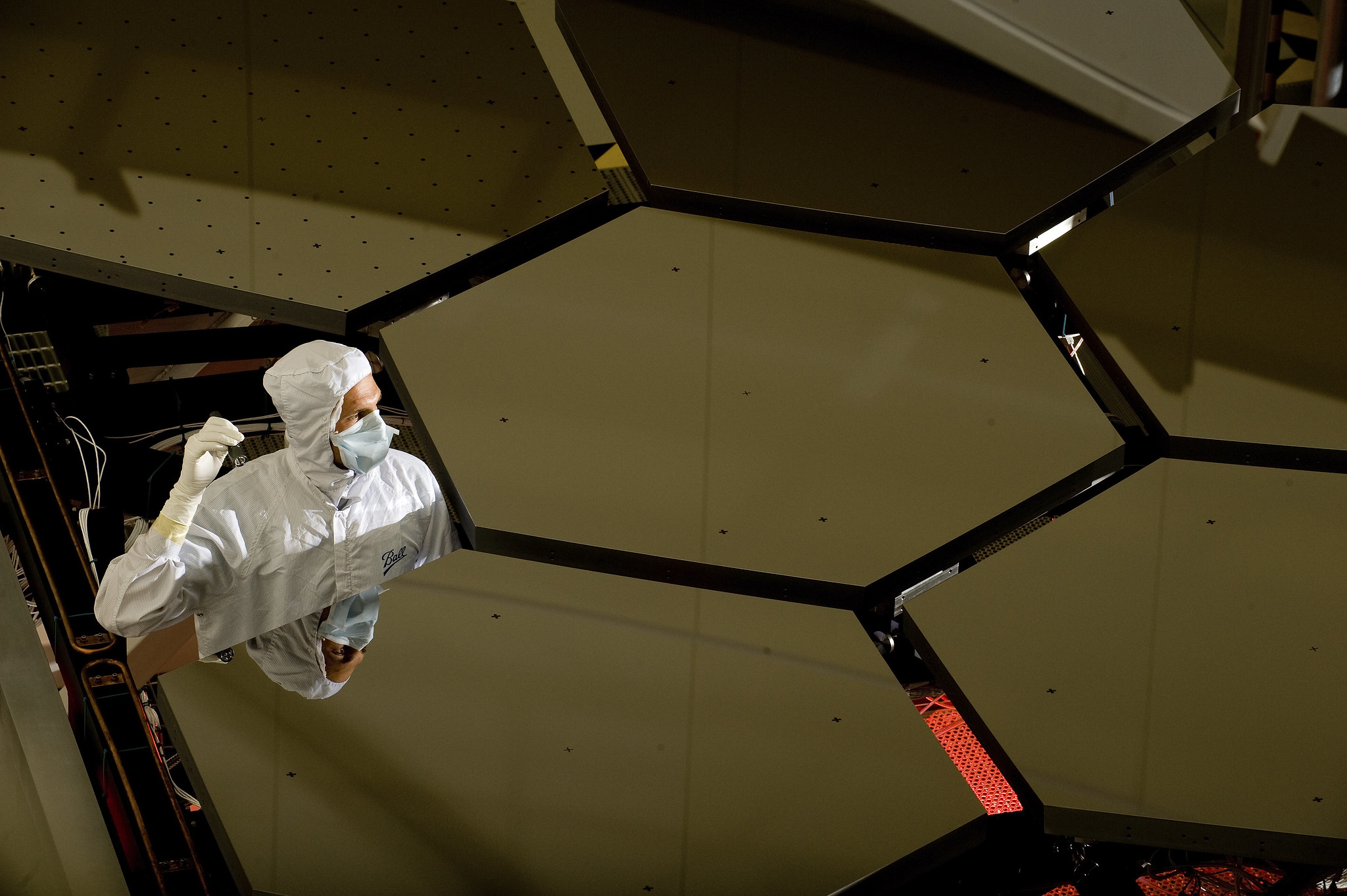
A technician inspects the Webb telescope's honeycomb-shaped mirror. The telescope's primary mirror is 21 feet (6.5 meters) across and is made up of 18 smaller hexagonal mirrors that must fold for launch and unfurl after the telescope reaches its orbit in space. Credit: NASA/MSFC/David Higginbotham/Emmett Given | › Full image and caption | + Expand image
Compared with the Hubble Space Telescope’s eight-foot (2.4 meter) diameter mirror, this gives the Webb telescope more than six times the surface area to collect those distant particles of light known as photons. Hubble’s famous Ultra Deep Field observation captured images of incredibly faint, distant galaxies by pointing at a seemingly empty spot in space for 16 days, but the Webb telescope will be able to make a similar observation in just seven hours.
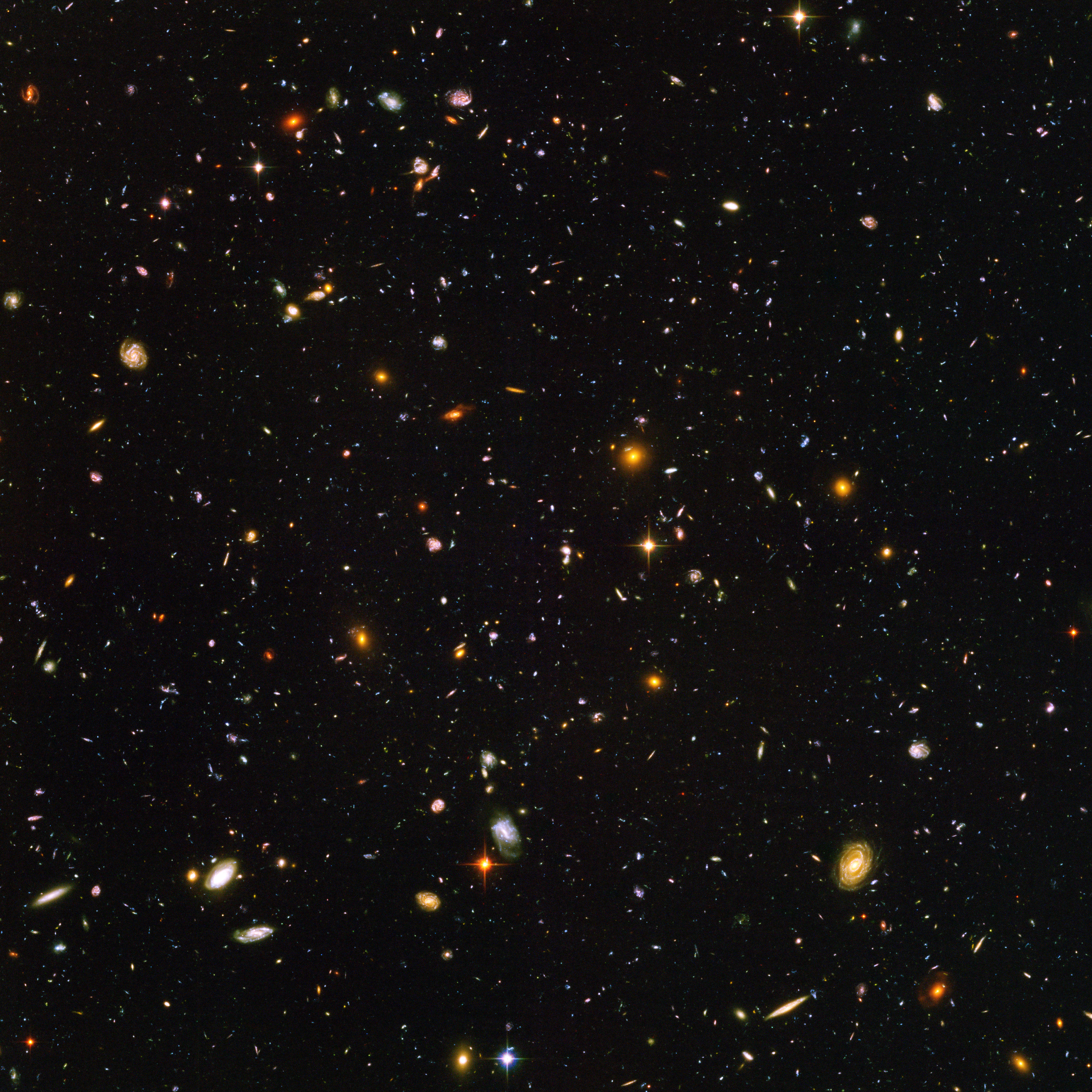
This image, called the Hubble Ultra Deep Field, shows 28 of the more than 500 young galaxies that existed when the universe was less than 1 billion years old. Credit: NASA, ESA, R. Bouwens and G. Illingworth (University of California, Santa Cruz) | › Full image and caption | + Expand image
Keeping Cool
The Webb Telescope gathers its scientific data as infrared light. To detect the faint signals of objects billions of light years away, the instruments inside the telescope have to be kept very cold, otherwise those infrared signals could get lost in the heat of the telescope. Engineers accounted for this with a couple of systems designed to get the instruments cold and keep them cold.
The Webb telescope's orbit around the Sun – sitting about 1 million miles (1.5 million kilometers) from Earth at Lagrange point 2 – keeps the spacecraft pretty far from our planet's heat, but even that’s not enough. To further reduce the temperature on the instruments, the spacecraft will unfurl a tennis-court-size sunshield that will block light and heat from the Sun, Earth, and Moon using five layers of specially coated material. Each layer blocks incoming heat, and the heat that does make it through is redirected out of the sides of the sunshield. Additionally, the vacuum between each layer provides insulation.

The sunshield is made up of five layers of specially coated material designed to block the Webb telescope's sensitive instruments from incoming heat from the Sun, Earth, and Moon. This photo, taken in the cleanroom at Northrop Grumman in Southern California in December 2020, shows the sunshield fully deployed and tensioned as it will be in space. Credit: NASA/Chris Gunn | › Full image and caption | + Expand image
The sunshield is so effective that the temperatures on the Sun-facing side of the telescope could be hot enough to boil water, while on the side closest to the instruments, the temperature could be as low as -394 F (-237 C, 36 K).
That’s cold enough for the near-infrared instruments to operate, but the Mid-Infrared Instrument, or MIRI, needs to be even colder. To bring down the temperature of MIRI, the Webb telescope is equipped with a special cryocooler that pumps chilled helium to the instrument to reduce its operating temperature to about -448 F (-267 C, 6 K).
Spotting Exoplanets
The Webb telescope will search for exoplanets using two different methods.
Using the transit method, the Webb telescope will look for the regular pattern of dimming that occurs when an exoplanet transits its star, or passes between the star and the telescope. The amount of dimming can tell scientists a lot about the passing exoplanet, such as the size of the planet and its distance from the star.

This animation shows how the transit method is used to hunt for planets outside our solar system. When exoplanets transit their parent star, the Webb telescope (like the Kepler space telescope, depicted here) will be able to detect the dip in the star’s brightness, providing scientists with key information about the transiting exoplanet. Students can see this technique in action with this transit math problem. Credit: NASA/JPL-Caltech | + Expand image
The second method the Webb telescope will use to search for exoplanets is direct imaging – capturing actual images of planets beyond our solar system. To enable direct imaging of exoplanets, the Webb telescope is equipped with a coronagraph. Just like you might use your hand to block a bright light, a coronagraph blocks starlight from reaching a telescope’s instruments, allowing a dim exoplanet orbiting a star to be seen.
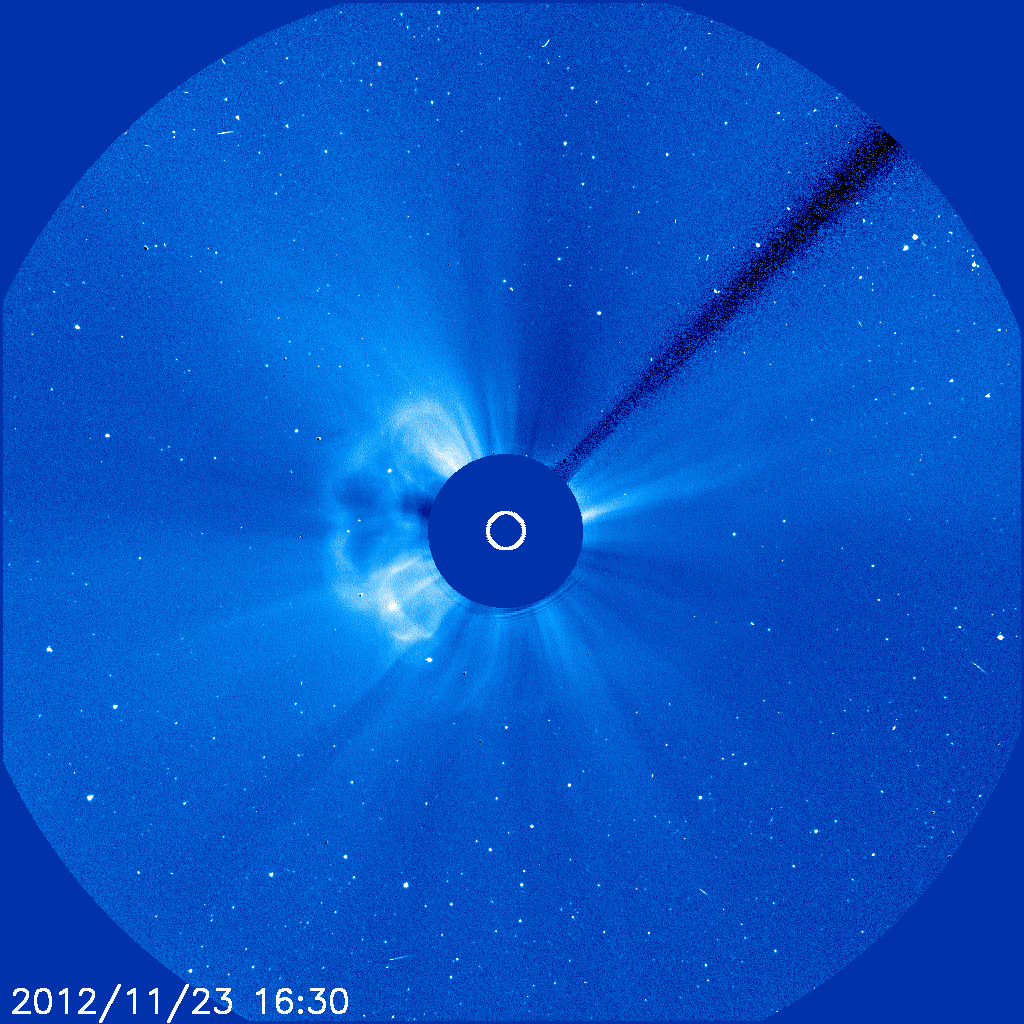
This “coronagraph” image taken by the Solar and Heliospheric Observatory, or SOHO, shows dim features around our Sun. Similarly, direct images of exoplanets captured by the Webb telescope will reveal details normally washed out by the brightness of stars. Credit: ESA&NASA/SOHO | › Full image and caption | + Expand image
The Webb telescope can uncover even more using spectroscopy. Light from a star produces a spectrum, which displays the intensity of light at different wavelengths. When a planet transits its star, some of the light from the star will pass through the planet's atmosphere before reaching the Webb telescope. Since all elements and molecules, such as methane and water, absorb energy at specific wavelengths, spectra from light that has passed through a planet’s atmosphere may contain dark lines known as absorption lines that tell scientists if there are certain elements present.
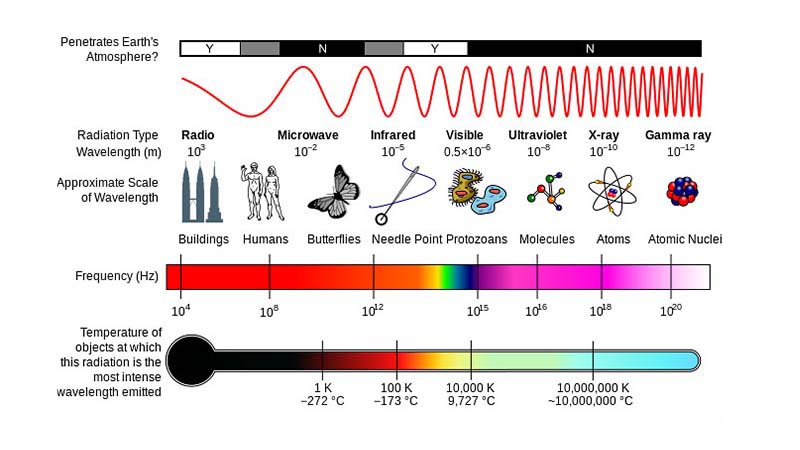
By looking at the unique spectrum produced when the light from a star shines through the atmosphere of a transiting exoplanet, scientists can learn whether certain elements are present on that planet. Credit: NASA | + Expand image
Using direct imaging and spectroscopy, scientists can learn even more about an exoplanet, including its color, seasons, rotation, weather, and vegetation if it exists.
All this could lead scientists to the ultimate exoplanet discovery: an Earth-size planet with an atmosphere like ours in its star’s habitable zone – a place where liquid water could exist.
Setting Up in Space
The Webb telescope will launch from French Guiana on top of an Ariane 5 rocket, a massive rocket capable of lifting the telescope, which weighs nearly 14,000 pounds (6,200 kilograms), to its destination.
The telescope's large mirror and giant sunshield are too big to fit inside the 18-foot (5.4-meter) wide rocket fairing, which protects the spacecraft during launch. To overcome this challenge, engineers designed the telescope's mirror and sunshield to fold for launch.
Two sides of the mirror assembly fold back for launch, allowing them to fit inside the fairing. The sunshield, which is 69.5 feet (21 meters) long and 46.5 feet (14 meters) wide, is carefully folded 12 times like origami so that it's narrow enough for launch. These are just two examples of several folding mechanisms needed to fit the massive telescope in its rocket for launch.
It will take about a month for the Webb telescope to reach its destination and unfurl its mirrors and sunshield. Scientists need another five months to cool down the instruments to their operating temperatures and align the mirrors correctly.
Approximately six months after launch, checkouts should be complete, and the telescope will begin its first science campaign and science operations.
Learn more and follow along with the mission from launch and unfolding to science observations and discovery announcements on the James Webb Space Telescope website.
Teach It
Check out these resources to bring the real-life STEM behind the mission into your teaching with lesson guides for educators, projects and slideshows for students, and more.
Educator Guides
Student Activities
Articles for Students
- What is the James Webb Space Telescope?
- What is the Big Bang?
- What is a galaxy?
- What is a satellite galaxy?
- What is a transit?
- What is a black hole?
- What is a light year?
- What is a nebula?
- What is an exoplanet?
- How many solar systems are in our galaxy?
- How old are galaxies?
- What is a supernova?
- Explore the electromagnetic spectrum
Videos for Students
- Space Place in a Snap: The Solar System’s Formation
- Space Place in a Snap: Searching for Other Planets Like Ours
Resources for Educators and Parents
Events
Explore More
- Mission Website: James Webb Space Telescope
- Photos: James Webb Space Telescope
- Videos: James Webb Space Telescope
- Facts & Figures: Mid-Infrared Instrument (MIRI)
NASA's Universe of Learning materials are based upon work supported by NASA under award number NNX16AC65A to the Space Telescope Science Institute, working in partnership with Caltech/IPAC, Center for Astrophysics | Harvard & Smithsonian, and the Jet Propulsion Laboratory.
TAGS: JWST, James Webb Space Telescope, electromagnetic spectrum, exoplanets, universe, solar system, big bang, cosmology, astronomy, star formation, galaxy, galaxies, telescope, life, technology, MIRI, Mars, Engineering, Teaching, Education, Classroom, Science, Universe of Learning