Teachable Moments | November 27, 2023
NASA Balloon Mission Designed to See the Space Between Stars
Get to know GUSTO and learn how to bring the science and engineering behind this unique balloon-based mission into the classroom.
A NASA balloon mission designed to study the interstellar medium – the space between stars – will take to the skies above Antarctica in December 2023.
Read on to learn how the GUSTO mission's unique design and science goals can serve as real-life examples of STEM concepts. Then, explore lessons and resources you can use to get students learning more.
What the GUSTO Mission Will Do
Though many people think of space as empty except for things like stars, planets, moons, asteroids, meteors, and comets, it’s anything but. Typically, there is one molecule of matter in every cubic centimeter of the space between stars known as the interstellar medium. In more dense clouds of interstellar gas, there could be as many as 1,000,000 molecules per cubic centimeter. It might not seem like much compared with the 10,000,000,000,000,000,000 molecules in every cubic centimeter of air we breathe, but the interstellar medium can tell us a lot about how stars and planets form and what role gases and dust play in our galaxy and others.

This diagram shows the life cycles of Sun-like and massive stars. Credit: NASA, Night Sky Network | › Learn more about star life cycles
Like plants and animals, stars have a life cycle that scientists want to better understand. Gases and dust grains that make up a dense interstellar cloud, known as a nebula, can become disturbed, and under the pull of their own gravity, begin collapsing in on themselves. Eventually stars form from the gas and planets form from the dust. As a star goes through its life, it eventually runs out of sources of energy. When this happens, the star dies, expelling gases – sometimes violently, as in a supernova – into a new gas cloud. From here, the cycle can start again. Scientists want to know more about the many factors at play in this cycle. This is where GUSTO comes in.
GUSTO – short for Galactic/Extragalactic ULDB Spectroscopic Terahertz Observatory – is a balloon-based telescope that will study the interstellar medium, the small amount of gas and dust between the stars. From its vantage point high above almost all of the Earth’s atmosphere, GUSTO will measure carbon, nitrogen, and oxygen emissions in the far-infrared portion of the electromagnetic spectrum, focusing its sights on the Milky Way galaxy and the nearby galaxy known as the Large Magellanic Cloud.

Our galaxy, the Milky Way, has hundreds of billions of stars and enough gas and dust to make billions more stars. Credit: NASA | › Full image and caption
The mission is designed to provide scientists with data that will help them understand the complete lifecycle of the gas and dust that forms planets and stars. To achieve its goals, GUSTO will study:
- The composition and formation of molecular clouds in these regions.
- The formation, birth, and evolution of stars from molecular clouds.
- The formation of gas clouds following the deaths of stars. And the re-start of this cycle.
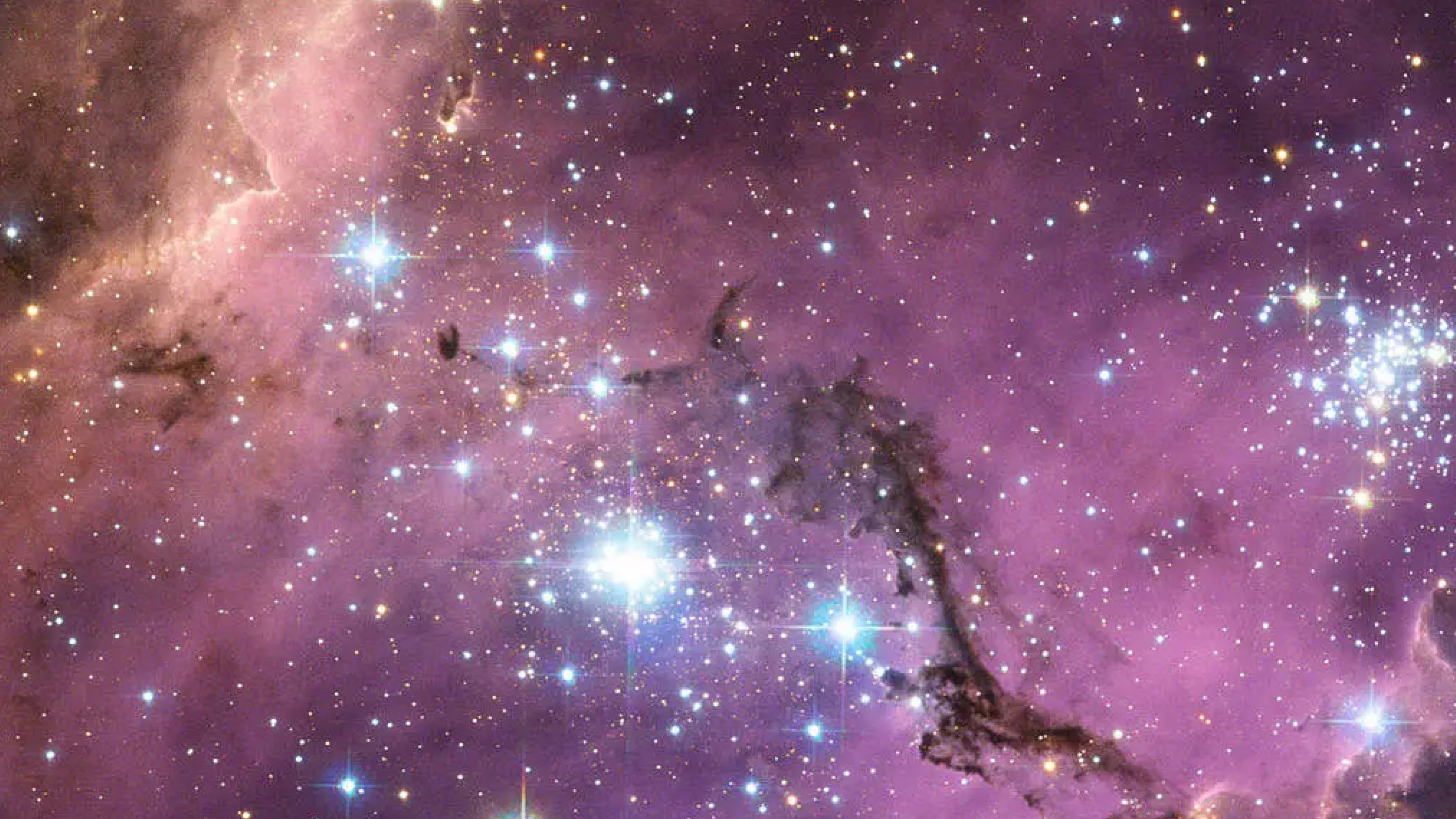
Nearly 200,000 light-years from Earth, the Large Magellanic Cloud is a satellite galaxy of the Milky Way. Vast clouds of gas within it slowly collapse to form new stars. In turn, these light up the gas clouds in a riot of colors, visible in this image from the Hubble Space Telescope. Credit: NASA | › Full image and caption
Scientists hope to use the information collected by GUSTO to develop models of the Milky Way and Large Magellanic Cloud. Studying these two galaxies allows scientists to observe more details and make more accurate models. Those models can then be used for comparing and studying more distant galaxies that are harder to observe.
Why Fly on a Balloon?
Unlike most NASA missions, GUSTO won’t launch on a rocket. It will be carried to approximately 120,000 feet (36.5 kilometers) above Antarctica using what’s known as a Long Duration Balloon, or LDB.
Balloon missions provide a number of advantages to scientists conducting research. They are more affordable than missions that go to space and require less time to develop. They also offer a way to test new scientific instruments and technologies before they are used in space. For these reasons, balloons have become a popular way for university students to gain experience building and testing science instruments.
Explore how balloons are being used for Earth and space science in this video from the Johns Hopkins Applied Physics Laboratory, which is providing the mission operations for GUSTO and the balloon gondola where the mission's instruments will be mounted. | Watch on YouTube
GUSTO's use of the Long Duration Balloon provided by NASA’s Balloon Science Program offers several advantages over other types of scientific balloons. Conventional scientific balloons stay aloft for a few hours or a few days and rely on the balloon maintaining a line-of-sight to send and receive data. Long Duration Balloons use satellites for sending data and receiving commands and can stay afloat for a few weeks to a couple of months.
Made with a thin, strong, plastic film called polyethylene, LDBs are partially inflated with helium. As the balloon rises, the surrounding air pressure decreases, allowing the gas inside the balloon to expand, increasing the volume and pressure of the balloon. When fully expanded, the balloon has a volume of around 40 million cubic feet (1.1 million cubic meters). That’s big enough to fit an entire football stadium inside.

GUSTO will be attached to a balloon gondola like the one depicted in this artist's rendering. | + Expand image
The telescope itself will be attached to a platform known as a gondola, which is home to several components that make the mission possible. The multi-axis control system will keep the platform stable during flight, allowing for precisely pointing GUSTO’s 35-inch (90-centimeter) diameter telescope in the right direction. Cryocoolers and liquid helium will keep the telescope’s scientific instruments at the necessary low temperature of -452°F (4° Kelvin). And the gondola will house a radio system that allows operators on the surface to control the balloon and telescope. All these systems will be powered by lithium-ion batteries charged during flight by a set of solar arrays.
Location is Everything
GUSTO is designed to measure terahertz wavelengths (in the far-infrared portion of the electromagnetic spectrum), a range of energy that is easily absorbed by water vapor. However, the observatory's altitude will put it in the upper half of the stratosphere and above 99% of the water vapor in the atmosphere. This makes it an ideal location for the mission to make its measurements and avoid factors that might otherwise obstruct its view.

GUSTO will make its observations from the upper half of the stratosphere, which offers several benefits over observing from lower in the atmosphere or from the ground. Credit: NASA | › Explore the interactive graphic
The stratosphere offers another advantage for GUSTO. This layer of the atmosphere warms as altitude increases, making the top of the stratosphere warmer than the bottom. The colder air at the bottom and warmer air at the top prevents mixing and air turbulence, making the air very stable and providing a great place to observe space. You may have noticed this stability if you’ve seen a flat-topped anvil-shaped storm cloud. That flat top is the cloud reaching the bottom of the stratosphere, where the stable air prevents the cloud from mixing upward.
But why fly GUSTO above Antarctica? Even though balloons can be launched from all over the planet, the 24 hours of sunlight per day provided by the Antarctic summer make the south polar region an ideal launch location for a solar-powered mission like GUSTO. But more important is a weather phenomenon known as an anticyclone. This weather system is an upper-atmosphere counter-clockwise wind flow that circles the South Pole about every two weeks. The Antarctic anticyclone allows for long balloon flights of missions that can be recovered and potentially reflown.
Preparing for Liftoff
To launch a balloon mission in Antarctica, weather conditions have to be just right. The anticyclone typically forms in mid-December but can arrive a little earlier or a little later. Even with the anticyclone started, winds on the ground and in the first few hundred feet of the atmosphere need to be under six knots (seven miles per hour) for GUSTO to launch. A NASA meteorologist provides daily updates on the cyclone and the ground.
Once weather conditions are good and the balloon is launched, it will circle Antarctica about once every 14 days with the wind. The anticyclone typically lasts one to two months. Because GUSTO may be in the air for more than two months, it’s possible that the mission will continue after the anticyclone ends, causing the balloon to drift northward as winter progresses.
Bring GUSTO Into the Classroom
The GUSTO mission is a great opportunity to engage students with hands-on learning opportunities. Students can build a planetary exploration balloon and model how interstellar dust forms into planets. Explore these lessons and resources to get students excited about the STEM involved in the mission.
Resources for Educators
- Lesson
Make a Planetary Exploration Balloon
In this engineering challenge, students must stay within design limitations while creating a balloon and gondola system that can descend or ascend at a given rate or maintain its altitude.
Subject Science
Grades 3-12
Time Less than 30 mins
- Lesson
The Science of Color
Quickly and easily model how colors reflect, absorb, and interact with each other in the classroom or online using your computer’s camera.
Subject Science
Grades 2-8
Time 30-60 mins
- Interactive
Star Formation: Eagle Nebula
View the Eagle Nebula in different wavelengths to see how new details emerge.
Subject Science
Resources for Students
- Project
Make a Planetary Exploration Balloon
Find out how NASA uses balloons to explore Earth and space and then take on a challenge to design your own balloon explorer inspired by what you've learned!
Subject Science
Grades 3-12
Time 30-60 mins
- Article
What Is a Galaxy?
Learn what galaxies are made of in this article from NASA Space Place.
- Article
How Old Are Galaxies?
Get the answer in this article from NASA Space Place.
- Interactive
Explore the Electromagnetic Spectrum
Click through this interactive from NASA Space Place all about the electromagnetic spectrum.
NASA's Universe of Learning materials are based upon work supported by NASA under award number NNX16AC65A to the Space Telescope Science Institute, working in partnership with Caltech/IPAC, Center for Astrophysics | Harvard & Smithsonian, and the Jet Propulsion Laboratory.
TAGS: GUSTO, Astronomy, Astrophysics, Science, Teaching, Learning, K-12, Classroom, Teachable Moments, Universe of Learning, Balloon Mission, Missions
Teacher Feature | April 3, 2023
Working With the Next Wave of Science Teachers
Four pre-service teachers at Cal Poly Pomona are developing their skills in lesson design and delivery as they study Earth science concepts and prepare for graduation.

Clockwise from upper left: Amie Gallardo, Sofia Vallejo, Afiya Kindle, Jacquelin Galvez-Coyt. Image courtesy: Brandon Rodriguez | + Expand image
During the fall semester of 2022, I had the privilege of working with the Education Department at California Polytechnic University in Pomona, specifically with pre-service teachers taking coursework in Earth science. During our collaboration, the curriculum had the students split time in class between learning about geology and Earth’s history and then designing and engaging in classroom activities related to the technical content that they could take to their own classes in the future. This combination had Cal Poly students learning science and education hand-in-hand each week and led to some amazing classroom lessons and lab activities.
One group of young women in the program stood out as exceptionally passionate about their future careers. This team consisted of four seniors: Jacquelin Galvez-Coyt, hoping to someday teach kindergarten; Amie Gallardo, who is planning to teach fourth grade; Afiya Kindle, who is interested in teaching elementary or middle school; and Sofia Vallejo, who is interested in kindergarten through sixth grade.
Despite their interest in working with young students and collaborating to design lessons for those students, each of these pre-service teachers allowed their individuality to shape how they navigated lesson design and implementation. I recently sat down with them to ask about their instructional style and aspirations for classrooms of their own.
Now that we’re back to in-person classes, how is the transition going?
Sofia: Returning from remote instruction felt eerie at first, but it’s so nice to return to communicate with people and build connections in a non-digital way. In-person classes prepare you to communicate with colleagues in real life, build social skills, and read body language. All of these skills are critical for a teacher in order to understand and better help students to succeed.
Amie: Returning from remote instruction has been amazing. While it had its perks, I believe, as students, we learn a lot more while working hands-on with our projects than is possible in distance learning. If we’re trying to develop and assess activities we can do with kids, that really requires being face-to-face.
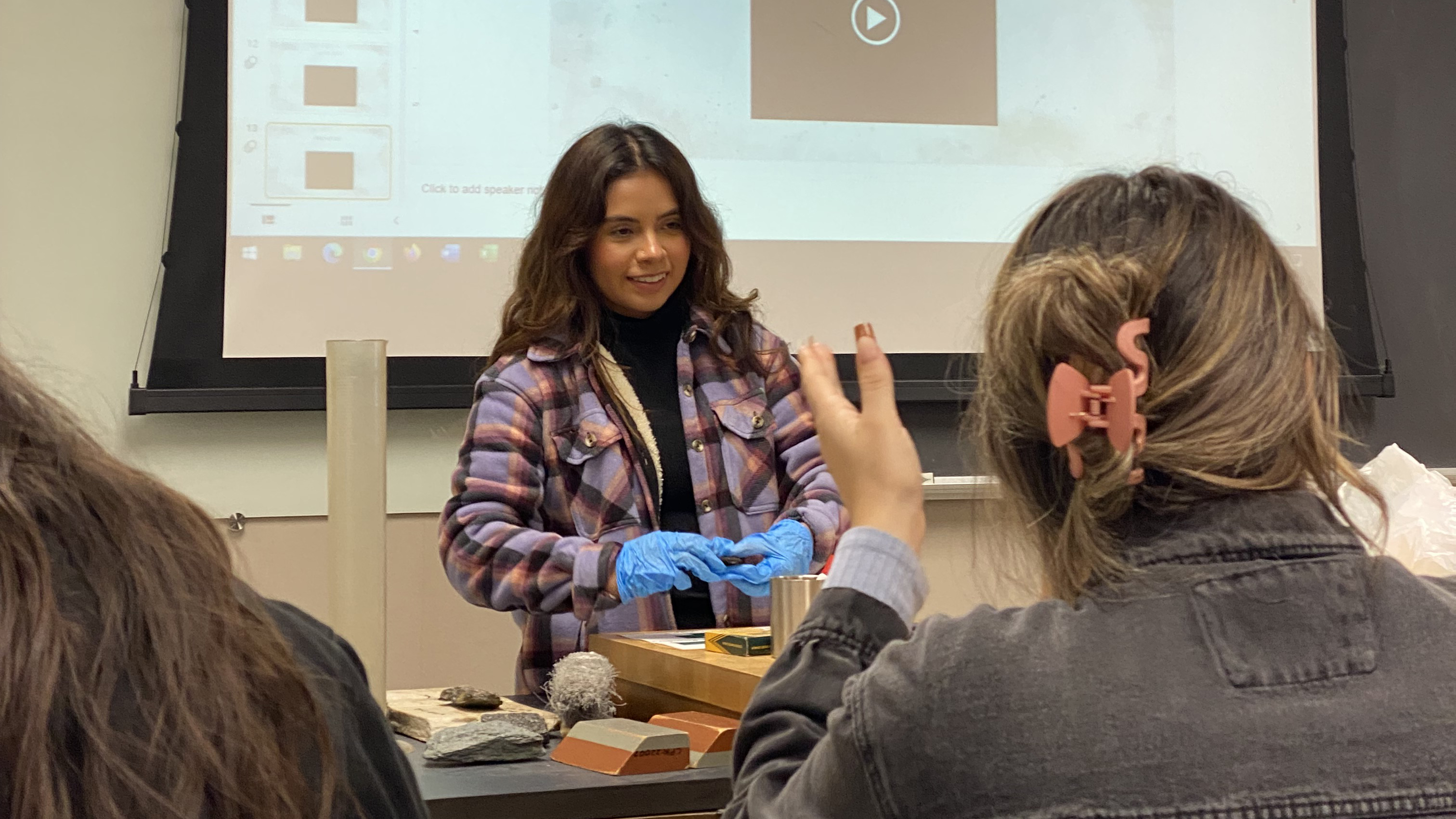
Amie Gallardo provides an Earth science demonstration to a class of education students at Cal Poly Pomona. Image courtesy: Brandon Rodriguez | + Expand image
What are you most excited about when it comes to having your own classroom, and how will you get your kids excited about STEM?
Afiya: I am most confident about creating a genuine safe space for kids. I’ll be able to communicate how much I care about them and about our shared future, and I think there could never be enough genuinely kind and caring teachers in this world.
Jacquelin: I think my kids will be excited about STEM because of how easy it's become to incorporate activities. There are many resources out there for teachers to use for teaching math and science that don't rely solely on a textbook. Activities that use inexpensive materials or that require a little DIY skills go a long way for students.
Afiya: Exactly! I know I developed my love for science from being hands-on and actually somewhat “in charge” of an experiment on my own. Winning a science fair competition in seventh grade for a greenhouse I built really boosted my confidence and helped reassure me of my scholastic abilities as a kid.
You led a really cool lesson with your classmates where you had them use Oreos to model tectonic boundaries. How do you feel that lesson went?
Jacqueline: I was really proud of our group. After giving a lecture to the students about tectonic plate boundaries, we dispersed Oreos to everyone. We were set up around the classroom demonstrating the activity and giving verbal instructions for everyone to follow. My favorite part was when I saw two students by me go, “Oohhhh,” and smile once they got their Oreos to demonstrate the plate boundaries correctly.
Amie: I thought it went really well! All the students in our classroom enjoyed it. Although we, as adults, may know about plate tectonics, having our hands on the Oreos to understand it made it more enjoyable.
Afiya: Plus, who doesn’t love Oreos? They’re even vegan!

An Oreo cookie is used to demonstrate rock fault movement. Image courtesy: Brandon Rodriguez | + Expand image
Which of the NASA-JPL lessons that you’ve implemented did you enjoy, and why?
Jacqueline: My favorite JPL activity we did was the Moon Phases activity. Having one team member to the side to give the instructions allows another student to view the different Moon phases. Then you switch so both students get to see that perspective. My second favorite activity was creating layers with different colored Play-Doh and demonstrating them as different plate boundaries and folds.
Amie: The NASA lesson that I enjoyed the most was the one we did on lunar eclipses. Much like myself, many students often have an early fascination with the Moon. Learning more about the Moon and lunar eclipses made me excited about the semester.
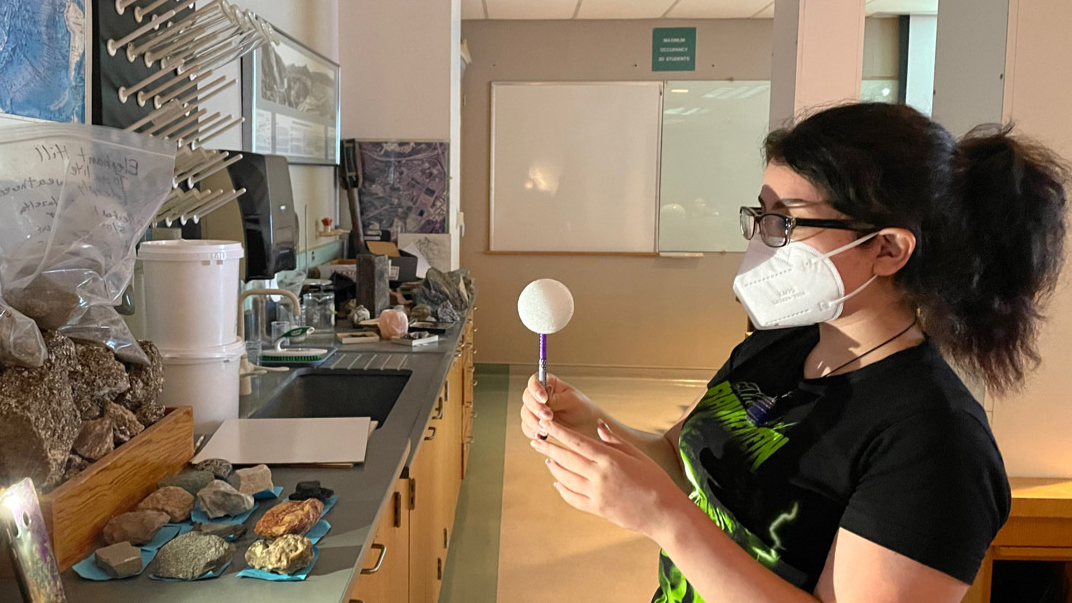
Sofia Vallejo uses a foam ball and lamp to demonstrate how solar eclipses occur. Image courtesy: Brandon Rodriguez | + Expand image
What’s next for you after you finish at Cal Poly Pomona?
Jacqueline: After I graduate at Cal Poly, I plan to attend UC Riverside to complete my credential program. While I am there, I would love to get my student teaching experience. Once I complete my credential program, I plan to apply to work at schools in the Inland Empire [in Southern California]. I want to be able to give back to the communities that influenced who I am today.
Sofia: My plans after Cal Poly are to take some time off to gain experience in the field as a substitute teacher. I also am looking to gain more volunteer experience, skills, and exposure. In the future, I want to enroll in UC Riverside to earn my teaching credential and master's degree.
Looking for ways to bring NASA STEM into your classroom or already have a great idea? The Education Office at NASA's Jet Propulsion Laboratory serves educators in the greater Los Angeles area. Contact us at education@jpl.nasa.gov.
Explore More
TAGS: Teachers, School, Remote School, Classroom, Instruction, K-12, STEAM, Science, Math, resources, lessons
Edu News | October 24, 2022
How Many Decimals of Pi Do We Really Need?
While world record holders may have memorized more than 70,000 digits of pi, a JPL engineer explains why you really only need a tiny fraction of that for most calculations – even at NASA.
Update: October 24, 2022 – This article, originally written in 2016, has been updated to reflect the latest values for NASA’s Voyager 1 spacecraft, which continues to venture farther into interstellar space. The author, Marc Rayman, has ventured on too, from the chief engineer for NASA’s Dawn mission, which concluded successfully in 2018, to the chief engineer for mission operations and science at NASA’s Jet Propulsion Laboratory.

This graphic shows more than 500 of the infinite number of decimals in pi. Image credit: NASA/JPL-Caltech | + Expand image
We received this question from a fan on Facebook who wondered how many decimals of the never-ending mathematical constant pi (π) NASA-JPL scientists and engineers use when making calculations:
“Does JPL only use 3.14 for its pi calculations? Or do you use more decimals, like say [360 or even more]?”
Here’s JPL’s Chief Engineer for Mission Operations and Science, Marc Rayman, with the answer:
Thank you for your question! This isn't the first time I've heard a question like this. In fact, it was posed many years ago by a sixth-grade science and space enthusiast who was later fortunate enough to earn a doctorate in physics and become involved in space exploration. His name was Marc Rayman.
To start, let me answer your question directly. For JPL's highest accuracy calculations, which are for interplanetary navigation, we use 3.141592653589793. Let's look at this a little more closely to understand why we don't use more decimal places. I think we can even see that there are no physically realistic calculations scientists ever perform for which it is necessary to include nearly as many decimal points as you asked about. Consider these examples:
- The most distant spacecraft from Earth is Voyager 1. As of this writing, it’s about 14.7 billion miles (23.6 billion kilometers) away. Let’s be generous and call that 15 billion miles (24 billion kilometers). Now say we have a circle with a radius of exactly that size, 30 billion miles (48 billion kilometers) in diameter, and we want to calculate the circumference, which is pi times the radius times 2. Using pi rounded to the 15th decimal, as I gave above, that comes out to a little more than 94 billion miles (more than 150 billion kilometers). We don't need to be concerned here with exactly what the value is (you can multiply it out if you like) but rather what the error in the value is by not using more digits of pi. In other words, by cutting pi off at the 15th decimal point, we would calculate a circumference for that circle that is very slightly off. It turns out that our calculated circumference of the 30-billion-mile (48-billion-kilometer) diameter circle would be wrong by less than half an inch (about one centimeter). Think about that. We have a circle more than 94 billion miles (more than 150 billion kilometers) around, and our calculation of that distance would be off by no more than the width of your little finger.
![]()
Put your pi math skills to the test with this problem from NASA's Pi Day Challenge. Can you use pi to determine what fraction of a signal from Voyager 1 reaches Earth? Image credit: NASA/JPL-Caltech | + Expand image | › View lesson page
- We can bring this closer to home by looking at our planet, Earth. It is more than 7,900 miles (12,700 kilometers) in diameter at the equator. The circumference is roughly 24,900 miles (40,100 kilometers). That's how far you would travel if you circumnavigated the globe – and didn't worry about hills, valleys, and obstacles like buildings, ocean waves, etc. How far off would your odometer be if you used the limited version of pi above? The discrepancy would be the size of a molecule. There are many different kinds of molecules, of course, so they span a wide range of sizes, but I hope this gives you an idea. Another way to view this is that your error by not using more digits of pi would be more than 30,000 times thinner than a hair!
![]()
Image credit: NASA | + Expand image
- Let's go to the largest size there is: the known universe. The radius of the universe is about 46 billion light years. Now let me ask (and answer!) a different question: How many digits of pi would we need to calculate the circumference of a circle with a radius of 46 billion light years to an accuracy equal to the diameter of a hydrogen atom, the simplest atom? It turns out that 37 decimal places (38 digits, including the number 3 to the left of the decimal point) would be quite sufficient. Think about how fantastically vast the universe is. It’s certainly far beyond what you can see with your eyes even on the darkest, most beautiful night of sparkling stars. It’s yet farther beyond the extraordinary vision of the James Webb Space Telescope. And the vastness of the universe is truly far, far, far beyond what we can even conceive. Now think about how incredibly tiny a single atom is. Isn’t it amazing that we wouldn’t need to use many digits of pi at all to cover that entire unbelievable range?
![]()
If you were to hold a single grain of sand at arm's length, you could cover the entire area of space taken up by this image, which was captured by the James Webb Space Telescope and contains thousands of galaxies. The oldest-known galaxy identified in the image is 13.1 billion years old. Image credit: NASA, ESA, CSA, STScI | + Expand image | › More about the image | Text description (PDF)
Pi is an intriguing number with interesting mathematical properties. It’s fun to think about its truly endless sequence of digits, and it may be surprising how often it appears in the equations scientists and engineers use. But there are no questions – prosaic or esoteric – in humankind’s noble efforts to explore or comprehend the marvels of the cosmos, from the unimaginably smallest scales to the inconceivably largest, that could require very many of those digits.
Hear more from Marc in his inspiring TEDx talk, “If It Isn’t Impossible, It Isn’t Worth Trying” and in his Dawn Journal, where he wrote frequent updates about the Dawn mission’s extraordinary extraterrestrial expedition to the protoplanet Vesta and dwarf planet Ceres.
Explore More
Educator Resources
-
NASA Pi Day Challenge
This collection of illustrated math problems gets students using pi like NASA scientists and engineers exploring Earth and space.
Grades 4-12
Time Varies
-
Pi in the Sky Lessons
Find everything you need to bring the NASA Pi Day Challenge into the classroom, including printable handouts of each illustrated math problem.
Grades 4-12
Time Varies
Articles
-
18 Ways NASA Uses Pi
Whether it's sending spacecraft to other planets, driving rovers on Mars, finding out what planets are made of or how deep alien oceans are, pi takes us far at NASA. Find out how pi helps us explore space.
-
10 Ways to Celebrate Pi Day With NASA on March 14
Find out what makes pi so special, how it’s used to explore space, and how you can join the celebration with resources from NASA.
Multimedia & Downloads
Recursos en español
TAGS: Pi, Pi Day, Dawn, Voyager, Engineering, Science, Mathematics
Teachable Moments | October 24, 2022
X-Ray Vision and Polarized Glasses Unite to Uncover Mysteries of the Universe
A NASA space telescope mission is giving astronomers a whole new way to peer into the universe, allowing us to uncover long-standing mysteries surrounding objects such as black holes. Find out how it works and how to engage students in the science behind the mission.
Some of the wildest, most exciting features of our universe – from black holes to neutron stars – remain mysteries to us. What we do know is that because of their extreme environments, some of these emit highly energetic X-ray light, which we can detect despite the vast distances between us and the source.
Now, a NASA space telescope mission is using new techniques to not only scout out these distant phenomena, but also provide new information about their origins. Read on to learn how scientists are getting exciting new perspectives on our universe and what the future of X-ray astronomy holds.
How They Did It
In 2021, NASA launched the Imaging X-Ray Polarimeter Explorer, or IXPE, through a collaboration with Ball Aerospace and the Italian Space Agency. The space telescope is designed to operate for two years, detecting X-rays emitted from highly energetic objects in space, such as black holes, different types of neutron stars (e.g., pulsars and magnetars) and active galactic nuclei. In its first year, the telescope is focusing on roughly a dozen previously studied X-ray sources, spending hours or even days observing each target to reveal new data made possible by spacecraft's scientific instruments.
IXPE isn't the first telescope to observe the universe in X-ray light. NASA's Chandra X-ray Observatory, launched in 1999, has famously spent more than 20 years photographing our universe at a wavelength of light exclusively found in high-energy environments, such as where cosmic materials are heated to millions of degrees as a result of intense magnetic fields or extreme gravity.
Using Chandra, scientists can assign colors to the different energy levels, or wavelengths, produced by these environments. This allows us to get a picture of the highly energetic light ejected by black holes and tiny neutron stars – small, but extremely dense stars with masses 10-25 times that of our Sun. These beautiful images, such as from Chandra’s first target, Cassiopeia A (Cas A for short), show the violent beauty of stars exploding.
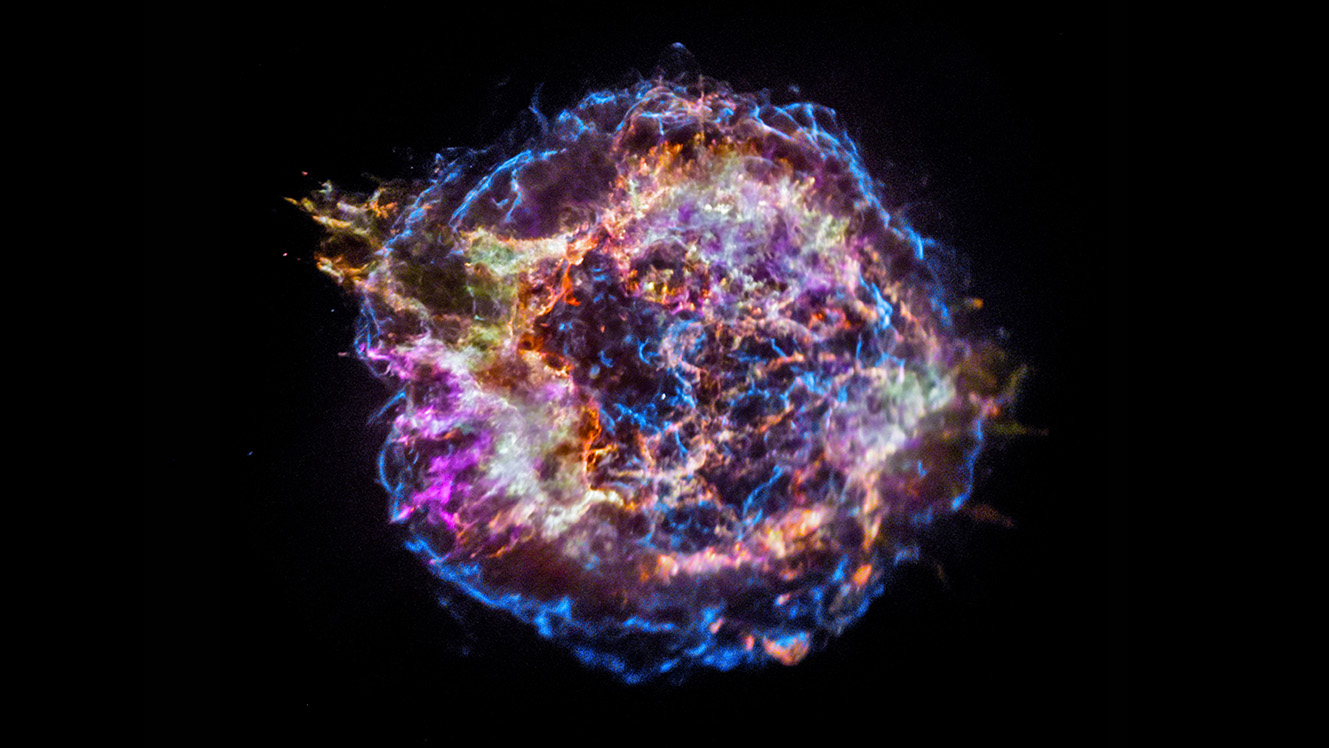
This image of the supernova Cassiopeia A from NASA’s Chandra X-ray Observatory shows the location of different elements in the remains of the explosion: silicon (red), sulfur (yellow), calcium (green) and iron (purple). Each of these elements produces X-rays within narrow energy ranges, allowing maps of their location to be created. Image credit: NASA/CXC/SAO | › Full image and caption
While Chandra has earned its name as one of “The Great Observatories,” astronomers have long desired to peer further into highly energetic environments in space by capturing them in even more detail.
IXPE expands upon Chandra’s work with the introduction of a tool called a polarimeter, an instrument used to understand the shape and direction of the light that reaches the space telescope's detectors. The polarimeter on IXPE allows scientists to gain insight into the finer details of black holes, supernovas, and magnetars, like which direction they are spinning and their three-dimensional shape.

This image of Cassiopeia A was created using some of the first X-ray data collected by IXPE, shown in magenta, combined with high-energy X-ray data from Chandra, in blue. Image credit: NASA/CXC/SAO/IXPE | › Full image and caption
While scientists have just begun putting IXPE's capabilities to use, they're already starting to reveal new details about the inner workings of these objects – such as the magnetic field environment around Cas A, shown in a newly released image.
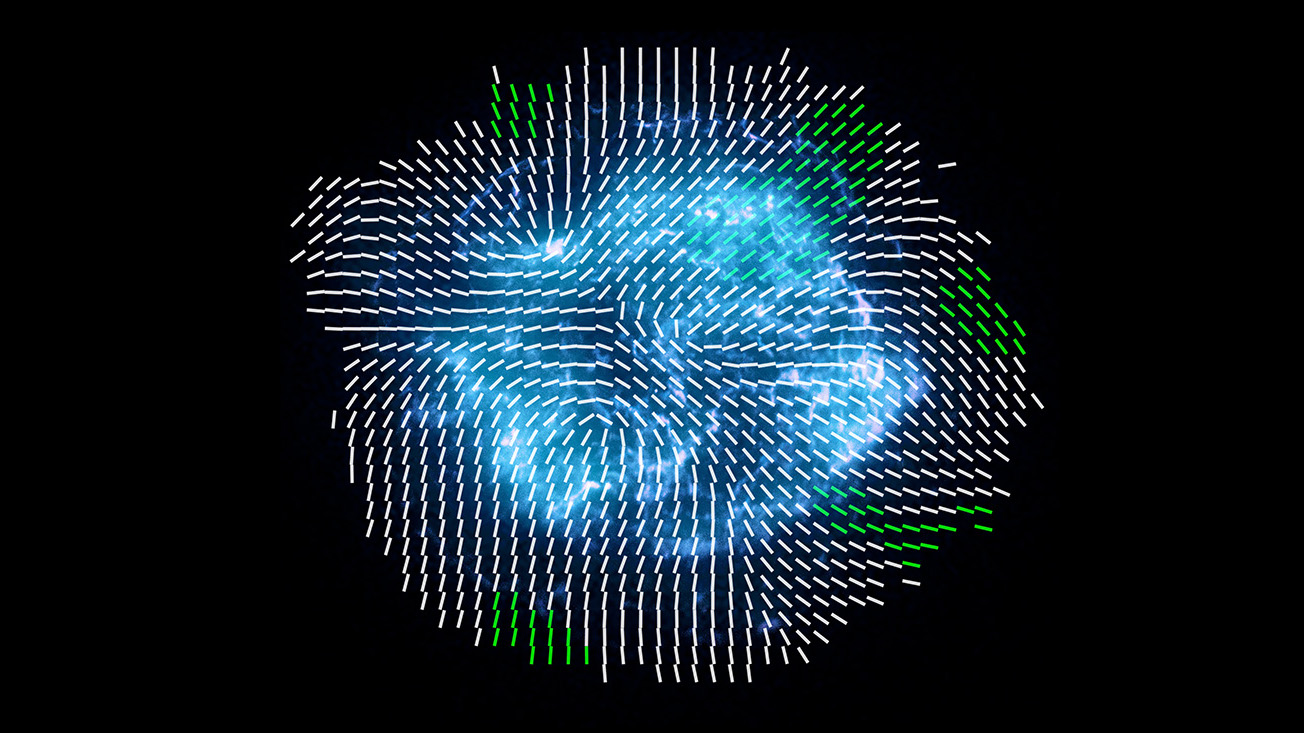
The lines in this newly released image come from IXPE measurements that show the direction of the magnetic field across regions of Cassiopeia A. Green lines indicate regions where the measurements are most highly significant. These results indicate that the magnetic field lines near the outskirts of the supernova remnant are largely oriented radially, i.e., in a direction from the center of the remnant outwards. The IXPE observations also reveal that the magnetic field over small regions is highly tangled, without a dominant preferred direction. Observations such as this one can help scientists learn how particles shooting out from supernovae interact with the magnetic field created by the explosion. Image credits: X-ray: Chandra: NASA/CXC/SAO; IXPE: NASA/MSFC/J. Vink et al. | + Expand image | › Full image and caption
“For the first time, we will use every collected photon of light to tell us about the nature and shapes of objects in the sky that would be dots of light otherwise,” says Roger Romani, a Stanford professor and the co-investigator on IXPE.
How It Works
Generally, when light is produced, it is what we call unpolarized, meaning that it oscillates in every direction. For example, our Sun produces unpolarized light. But sometimes, light is produced in a highly organized fashion, oscillating only in one direction. In astronomy, this arises when magnetic fields force particles to incredibly high speeds, creating highly organized, or polarized, light.
This is what makes objects like the supernova Cas A such enticing targets for IXPE. Exploded stars like Cas A generate massive energetic waves when they go supernova, giving scientists a view of how particles shooting out at immense speeds interact with the magnetic fields from such an event. In the case of Cas A, IXPE was able to determine that the x-rays are not very polarized, meaning the explosion created very turbulent regions with multiple field directions.
While the idea of polarized or organized light may sound abstract, you may have noticed it the last time you were outside on a sunny day. If you’ve tried on a pair of polarized sunglasses, you may have noticed that the glare was greatly reduced. That’s because as light scatters, it bounces off of reflective surfaces in all directions. However, polarized lenses have tiny filters that only allow light coming from a narrow band of directions to pass through.
The polarimeter on IXPE works in a similar way. Astronomers can determine the strength of an object's magnetic field by using the polarimeter to measure how much of the light detected by the telescope is polarized. Typically, the more polarized the light the stronger the magnetic field at the source.
Astronomers can even go a step further to measure the direction this light is oscillating by measuring the angle of the light that reaches the telescope. Because the polarized light leaves the source in a predictable fashion – namely perpendicular from its magnetic field – knowing the angle of the oscillating light provides information about the axis of rotation and potentially even the surface structure of objects such as neutron stars and nebulae.

In this demonstration, the rope represents light waves and the open window represents a polarimeter. Depending on the angle of the light waves (rope), more or less information makes it through the polarimeter (window) the narrower it is. By measuring the amount of light received through the polarimeter, IXPE can determine the angle and the polarization of the light. Image credit: NASA/JPL-Caltech | + Expand image
Imagine, for example, that you were holding one end of a piece of rope secured to an object at the other end. If you swung the rope side to side to make horizontal waves, those waves would be able to make it through a narrow target like a window. If you started to shut the window from the top, narrowing the opening, the waves could conceivably still make it through the opening. However, if you made veritcal waves by waving the rope up and down, as the window closed, fewer and fewer waves would make it through the opening. Likewise, by measuring the light that makes it through the polarimeter to the detector on the other side, IXPE can determine the angle of the light received.
To collect this light, IXPE uses three identical mirrors at the end of a four meter (13 foot) boom. The light received by IXPE is carefully focused on the spacecraft’s polarimeter at the other end of the boom, allowing scientists to collect those crucial measurements.
During the IXPE launch broadcast, commentators discuss the components of the spacecraft and how it measures polarization. | Watch on YouTube
Why It's Important
Building on Chandra's observations from the past two decades, IXPE's novel approach to X-ray science is pulling the curtain back even farther on some of the most fascinating objects in the universe, providing first looks at how and where radiation is being produced in some of the most extreme environments in the universe. IXPE's measurements of Cas A are just the beginning, with even more mysterious targets ready to be explored.
Take it from Martin Weisskopf, the principal scientist on IXPE and project scientist for Chandra, who has spent his 50-year career working in X-ray astronomy, who says, “IXPE will open up the field in ways we’ve been stuck only theorizing about."
Teach It
Explore more on how NASA uses light to map our universe, and dig deeper into some of the celestial features it allows to study, such as blackholes and neutron stars.
Activities
- Project
Exploring Materials - Polarizers
In this activity, learners experiment how polarizers affect light by using two polarizing sheets and overlapping layers of transparent tape.
- Activity Guidebook
Girls STEAM Ahead with NASA Program Cookbook
A guidebook for facilitators planning their own Girls STEAM Ahead with NASA event using NASA’s Universe of Learning resources.
- Slideshow
Black Holes: By the Numbers
What are black holes and how do they form? Explore more in this slideshow.
- Video
What Is a Black Hole?
Find out how what a black hole is, how they can form and why they are so cool!
Educator Resources
-
Science Briefing: Exploring the High Energy Universe
Explore the event materials from this science briefing to get a window into the most energetic processes and the most extreme objects in the universe.
Subject Science
-
The Science of Color
Quickly and easily model how colors reflect, absorb, and interact with each other in the classroom or online using your computer’s camera.
Subject Science
Grades 2-8
Time < 30 mins
-
Using Light to Study Planets
Students build a spectrometer using basic materials as a model for how NASA uses spectroscopy to determine the nature of elements found on Earth and other planets.
Subject Science
Grades 6-11
Time 2+ hrs
-
Dropping In With Gravitational Waves
Students develop a model to represent the collision of two black holes, the gravitational waves that result and the waves' propagation through spacetime.
Subject Science
Grades 6-8
Time 30-60 mins
- Teachable Moments
Telescopes Get Extraordinary View of Milky Way's Black Hole
Find out how scientists captured the first image of Sagittarius A*, why it's important, and how to turn it into a learning opportunity for students.
- Teachable Moments
How Scientists Captured the First Image of a Black Hole
Find out how scientists created a virtual telescope as large as Earth itself to capture the first image of a black hole's silhouette.
- Teachable Moments
Gravitational Waves Detected for the First Time
Find out how researchers proved part of Albert Einstein’s Theory of General Relativity, then create a model of the Nobel Prize-winning experiment in the classroom.
Explore More
- Article: NASA’S IXPE Helps Unlock the Secrets of Famous Exploded Star
- Educator Guide: Black Hole Math
- Opportunity: NASA/IPAC TeacherArchive Research Program
- Student Resources: Chandra
- Article: Hubble - Black Holes
- Interactive: Sagittarius A*
- Video: Hubble - Black Holes
- Website: NASA Science - Black Holes
- Download: A Galaxy Full of Black Holes Presentation
- Expert Talk: Imaging a Black Hole Lecture
- Facts & Figures: NASA - Black Holes
- Article: Black Hole Image Makes History
- Infographic: Anatomy of a Black Hole
NASA's Universe of Learning materials are based upon work supported by NASA under award number NNX16AC65A to the Space Telescope Science Institute, working in partnership with Caltech/IPAC, Center for Astrophysics | Harvard & Smithsonian, and the Jet Propulsion Laboratory.
TAGS: Universe, Stars and Galaxies, Space Telescope, IXPE, Astronomy, Science, Electromagnetic Spectrum, Universe of Learning
Teachable Moments | October 20, 2022
The Science Behind NASA's First Attempt at Redirecting an Asteroid
Find out more about the historic first test, which could be used to defend our planet if a hazardous asteroid were discovered. Plus, explore lessons to bring the science and engineering of the mission into the classroom.
Update: Oct. 20, 2022 – The DART spacecraft successfully impacted the asteroid Dimorphos on September 26, reducing the period of the asteroid's orbit by 32 minutes. Scientists considered a change of 73 seconds to be the minimum amount for success. This article has been updated to reflect the latest data and images from the impact.
In a successful attempt to alter the orbit of an asteroid for the first time in history, NASA crashed a spacecraft into the asteroid Dimorphos on Sept. 26, 2022. The mission, known as the Double Asteroid Redirection Test, or DART, took place at an asteroid that posed no threat to our planet. Rather, it was an ideal target for NASA to test an important element of its planetary defense plan.
Read further to learn about DART, how it worked, and how the science and engineering behind the mission can be used to teach a variety of STEM topics.
Why It's Important
The vast majority of asteroids and comets are not dangerous, and never will be. Asteroids and comets are considered potentially hazardous objects, or PHOs, if they are 100-165 feet (30-50 meters) in diameter or larger and their orbit around the Sun comes within five million miles (eight million kilometers) of Earth’s orbit. NASA's planetary defense strategy involves detecting and tracking these objects using telescopes on the ground and in space. In fact, NASA’s Center for Near Earth Object Studies, or CNEOS, monitors all known near-Earth objects to assess any impact risk they may pose. Any relatively close approach is reported on the Asteroid Watch dashboard.
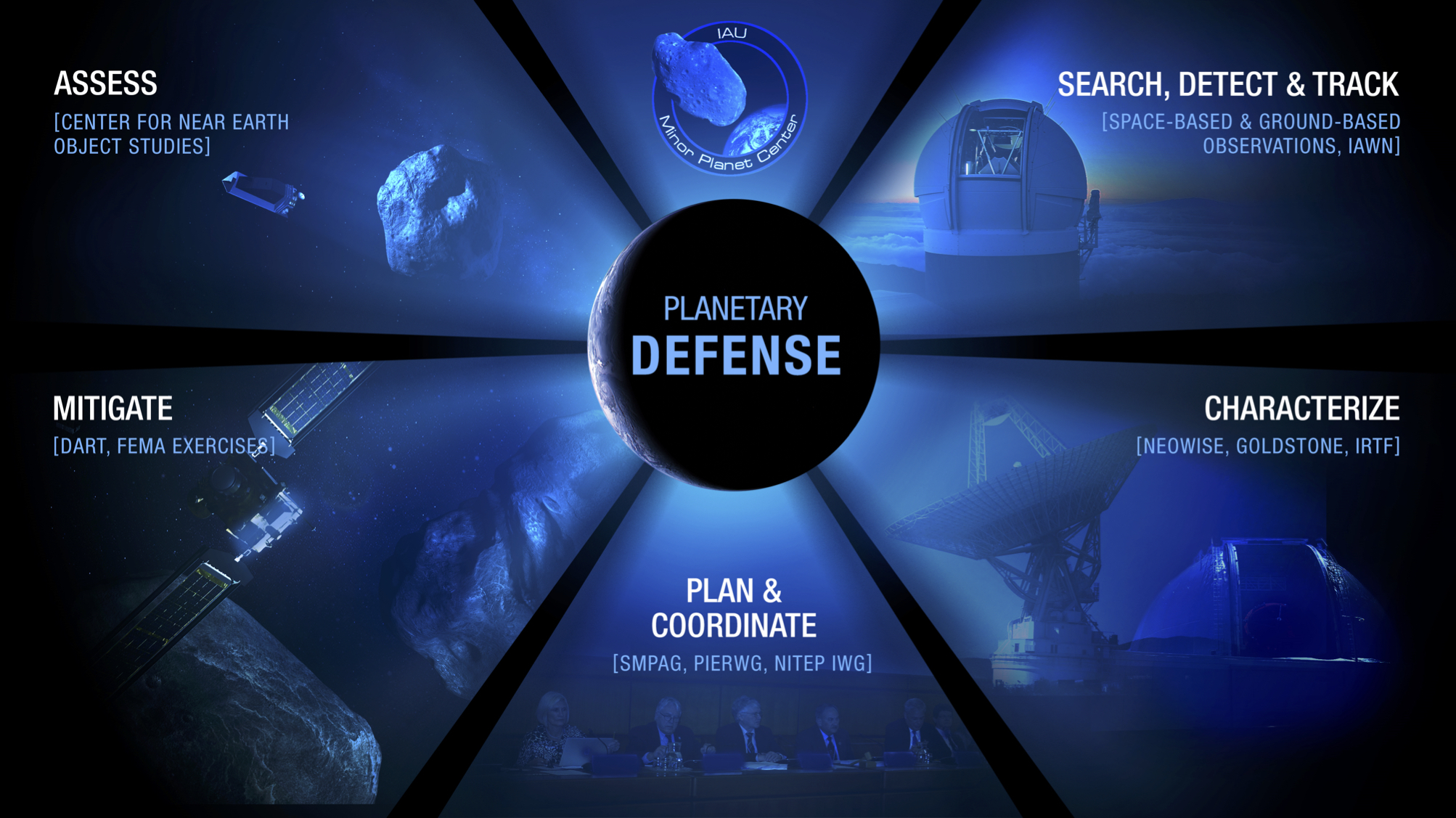
NASA's Planetary Defense Coordination Office runs a variety of programs and initiatives aimed at detecting and responding to threats from potentially hazardous objects, should one be discovered. The DART mission is one component and the first mission being flown by the team. Image credit: NASA | + Expand image
While there are no known objects currently posing a threat to Earth, scientists continue scanning the skies for unknown asteroids. NASA is actively researching and planning for ways to prevent or reduce the effects of a potential impact, should one be discovered. The DART mission was the first test of such a plan – in this case, whether it was possible to divert an asteroid from its predicted course by slamming into it with a spacecraft.
Eyes on Asteroids is a real-time visualization of every known asteroid or comet that is classified as a near-Earth object, or NEO. Asteroids are represented as blue dots and comets as shown as white dots. Use your mouse to explore the interactive further and learn more about the objects and how we track them. Credit: NASA/JPL-Caltech | Explore the full interactive
With the knowledge gained from the demonstration, similar techniques could be used in the future to deflect an asteroid or comet away from Earth if it were deemed hazardous to the planet.
How It Worked
With a diameter of about 525 feet (160 meters) – the length of 1.5 football fields – Dimorphos is the smaller of two asteroids in a double-asteroid system. Before DART's impact, Dimorphos orbited the larger asteroid called Didymos (Greek for "twin"), every 11 hours and 55 minutes.
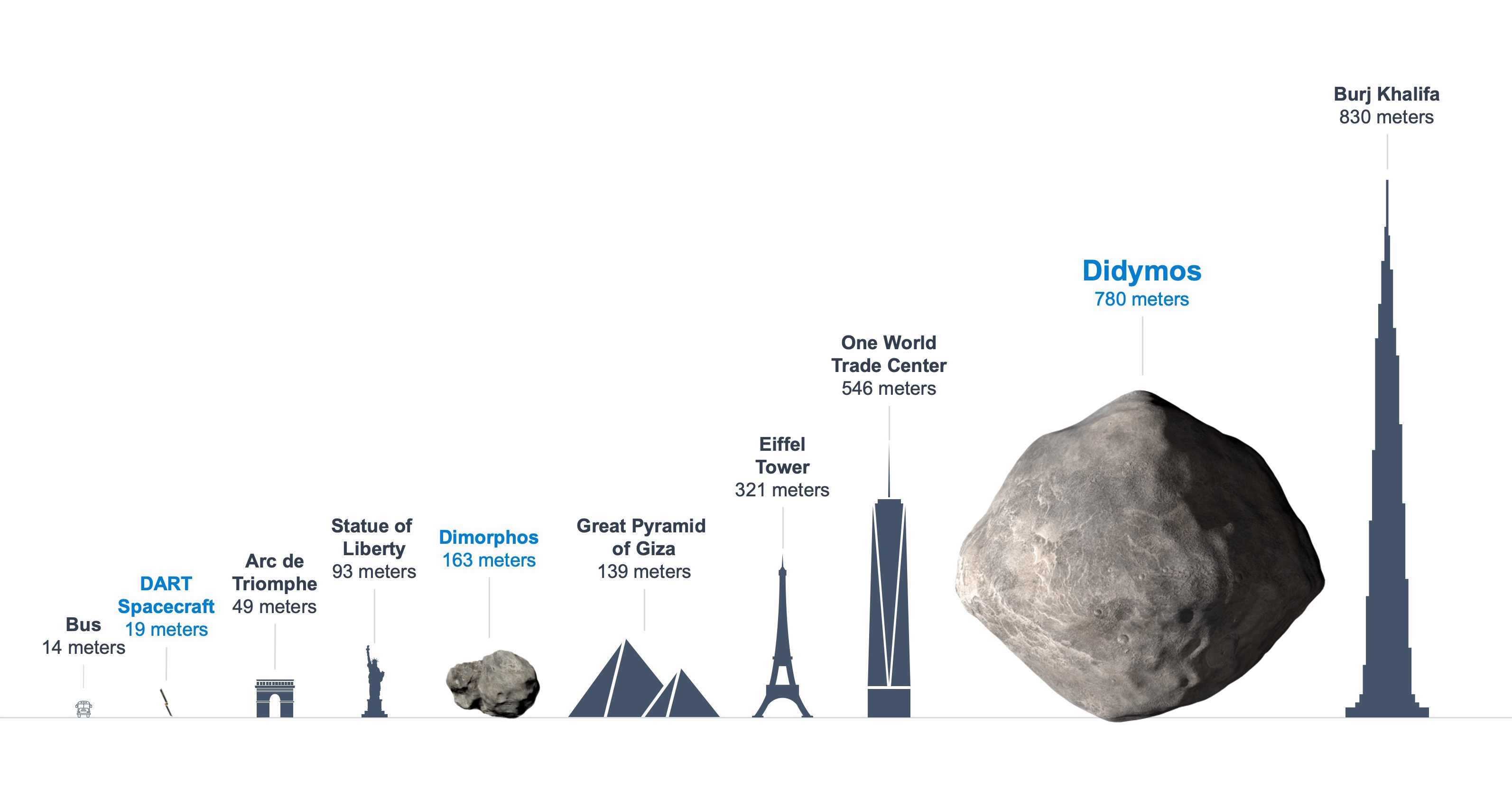
The sizes of the two asteroids in the Didymos system relative to objects on Earth. Image credit: NASA/Johns Hopkins APL | + Expand image
Neither asteroid poses a threat to our planet, which is one reason why this asteroid system was the ideal place to test asteroid redirection techniques. At the time of DART's impact, the asteroid pair was 6.8 million miles (11 million kilometers) away from Earth as they traveled on their orbit around the Sun.
The DART spacecraft was designed to collide head-on with Dimorphos to alter its orbit, shortening the time it takes the small asteroid to travel around Didymos. Compared with Dimorphos, which has a mass of about 11 billion pounds (five billion kilograms), the DART spacecraft was light. It weighed just 1,210 pounds (550 kilograms) at the time of impact. So how did such a light spacecraft affect the orbit of a relatively massive asteroid?
You can use your mouse to explore this interactive view of DART's impact with Dimorphos from NASA's Eyes on the Solar System. Credit: NASA/JPL-Caltech | Explore the full interactive
DART was designed as a kinetic impactor, meaning it transferred its momentum and kinetic energy to Dimorphos upon impact, altering the asteroid's orbit in return. Scientists were able to make predictions about some of these effects thanks to principles described in Newton's laws of motion.
Newton’s first law told us that the asteroid’s orbit would remain unchanged until something acted upon it. Using the formula for linear momentum (p = m * v), we could calculate that the spacecraft, which at the time of impact would be traveling at 3.8 miles (6.1 kilometers) per second, would have about 0.5% of the asteroid’s momentum. The momentum of the spacecraft may seem small in comparison, but calculations suggested it would be enough to make a detectable change in the speed of Dimorphos' orbit. However, mission planners felt that changing Dimorphos’ orbit by at least 73 seconds would be enough to consider the test a success.
But there was more to consider in testing whether the technique could be used in the future for planetary defense. For example, the formula for kinetic energy (KE = 0.5 * m * v2) tells us that a fast moving spacecraft possesses a lot of energy.
When DART hit the surface of the asteroid, its kinetic energy was 10 billion joules! A crater was formed and material known as ejecta was blasted out as a result of the impact. Scientists are still studying the data returned from the mission to determine the amount of material ejected out of the crater, but estimates prior to impact put the number at 10-100 times the mass of the spacecraft itself. The force needed to push this material out was then matched by an equal reaction force pushing on the asteroid in the opposite direction, as described by Newton’s third law.
This animation shows conceptually how DART's impact is predicted to change Dimorphos' orbit from a larger orbit to a slightly smaller one that's several minutes shorter than the original. Credit: NASA/Johns Hopkins APL/Jon Emmerich | Watch on YouTube
How much material was ejected and its recoil momentum is still unknown. A lot depends on the surface composition of the asteroid, which scientists are still investigating. Laboratory tests on Earth suggested that if the surface material was poorly conglomerated, or loosely formed, more material would be blasted out. A surface that was well conglomerated, or densely compacted, would eject less material.
After the DART impact, scientists used a technique called the transit method to see how much the impact changed Dimorphos' orbit. As observed from Earth, the Didymos pair is what’s known as an eclipsing binary, meaning Dimorphos passes in front of and behind Didymos from our view, creating what appears from Earth to be a subtle dip in the combined brightness of the pair. Scientists used ground-based telescopes to measure this change in brightness and calculate how quickly Dimorphos orbits Didymos. By comparing measurements from before and after impact, scientists determined that the orbit of Dimorphos had slowed by 32 minutes to 11 hours and 23 minutes.
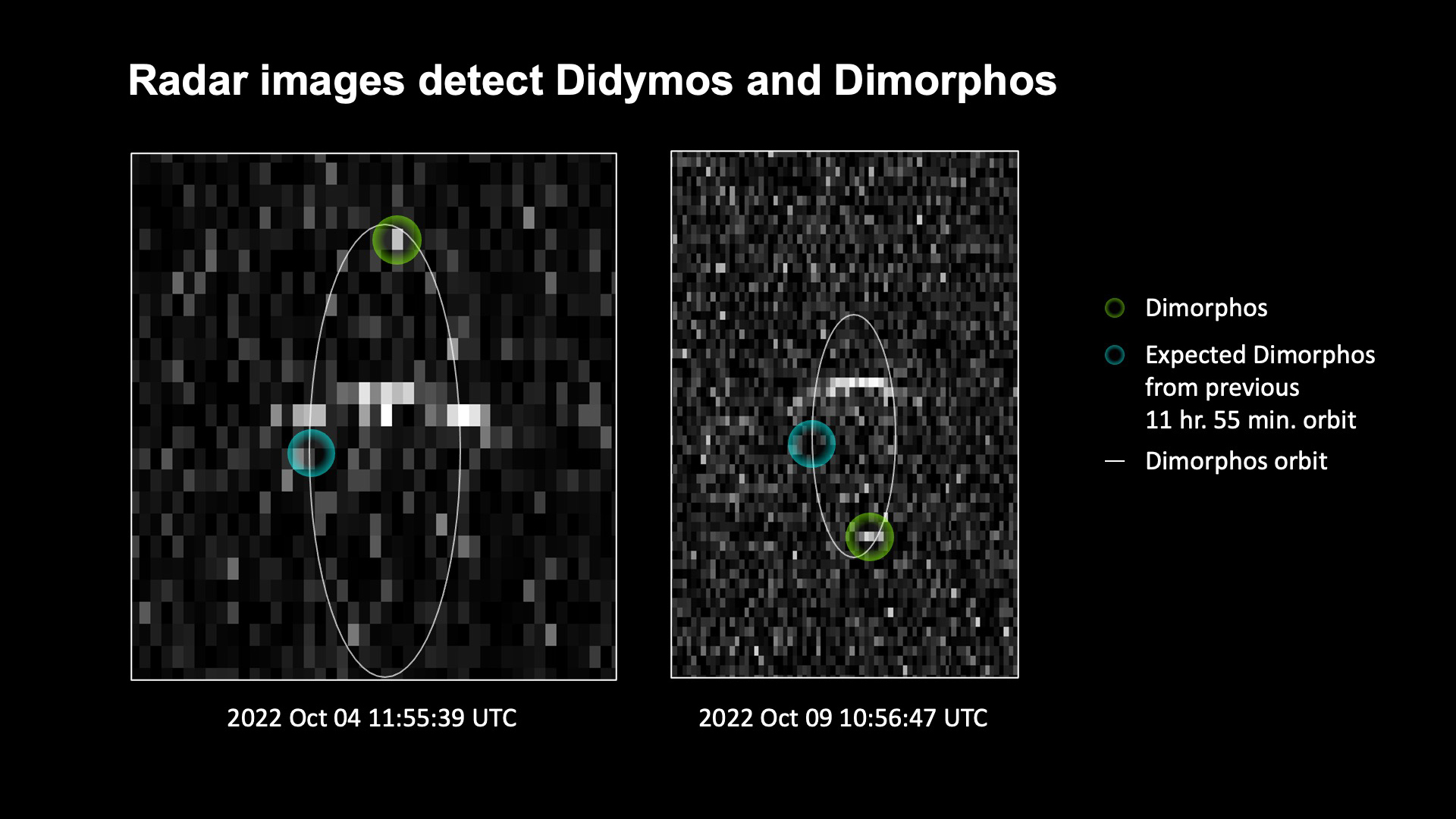
The green circle shows the location of the Dimorphos asteroid, which orbits the larger asteroid, Didymos, seen here as the bright line across the middle of the images. The blue circle shows where Dimorphos would have been had its orbit not changed due to NASA’s DART mission purposefully impacting the smaller asteroid on Sept. 26, 2022. The images were obtained from the NASA Jet Propulsion Laboratory’s Goldstone planetary radar in California and the National Science Foundation’s Green Bank Observatory in West Virginia. Image credit: NASA/Johns Hopkins APL/JPL/NASA JPL Goldstone Planetary Radar/National Science Foundation’s Green Bank Observatory | + Expand image | › DART image gallery
One of the biggest challenges of the DART mission was navigating a small spacecraft to a head-on collision with a small asteroid millions of miles away. To solve that problem, the spacecraft was equipped with a single instrument, the DRACO camera, which worked together with an autonomous navigation system called SMART Nav to guide the spacecraft without direct control from engineers on Earth. About four hours before impact, images captured by the camera were sent to the spacecraft's navigation system, allowing it to identify which of the two asteroids was Dimorphos and independently navigate to the target.

A composite of 243 images of Didymos and Dimorphos taken by the DART spacecraft's DRACO camera on July 27, 2022, as the spacecraft was navigating to its target. Image credit: JPL DART Navigation Team | + Expand image | › DART image gallery
DART was not just an experimental asteroid impactor. The mission also used cutting-edge technology never before flown on a planetary spacecraft and tested new technologies designed to improve how we power and communicate with spacecraft.
Learn more about the engineering behind the DART mission, including the innovative Roll Out Solar Array and NEXT-C ion propulsion system, in this video featuring experts from the mission. Credit: APL | Watch on YouTube
One such technology that was first tested on the International Space Station and was later used on the solar-powered DART spacecraft, is the Roll Out Solar Array, or ROSA, power system. As its name suggests, the power system consisted of flexible solar panel material that was rolled up for launch and unrolled in space.

The Roll Out Solar Array, shown in this animated image captured during a test on the International Space Station, is making its first planetary journey on DART. Image credit: NASA | + Expand image
Some of the power generated by the solar array was used for another innovative technology, the spacecraft's NEXT-C ion propulsion system. Rather than using traditional chemical propulsion, DART was propelled by charged particles of xenon pushed from its engine. Ion propulsion has been used on other missions to asteroids and comets including Dawn and Deep Space 1, but DART's ion thrusters had higher performance and efficiency.
Follow Along
In the days following the event, NASA received images of the impact from a cubesat, LICIACube, that was deployed by DART before impact. The cubesat, which was provided by the Italian Space Agency, captured images of the impact and the ejecta cloud.
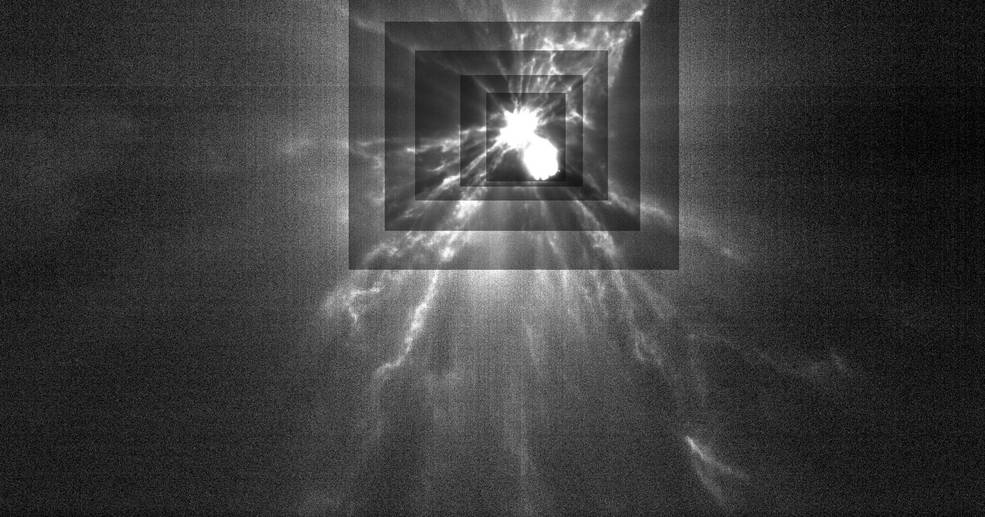
This image from LICIACube shows plumes of ejecta streaming from Dimorphos after DART's impact. Each rectangle represents a different level of contrast to better see fine structure in the plumes. By studying these streams of material, scientists will be able to learn more about the asteroid and the impact process. | + Expand image | › DART image gallery
Meanwhile, the James Webb Space Telescope, the Hubble Space Telescope, and the Lucy spacecraft observed Didymos to monitor how soon reflected sunlight from the ejecta plume could be seen. Going forward, DART team members will continue observing the asteroid system to measure the change in Dimorphos’ orbit and determine what happened on its surface. And in 2024, the European Space Agency plans to launch the Hera spacecraft to conduct an in-depth post-impact study of the Didymos system.

This animation, a timelapse of images from NASA’s James Webb Space Telescope, covers the time spanning just before DART's impact at 4:14 p.m. PDT (7:14 p.m. EDT) on Septtember 26 through 5 hours post-impact. Plumes of material from a compact core appear as wisps streaming away from where the impact took place. An area of rapid, extreme brightening is also visible in the animation. Image credit: Science: NASA, ESA, CSA, Cristina Thomas (Northern Arizona University), Ian Wong (NASA-GSFC); Joseph DePasquale (STScI) | + Expand image | › DART image gallery
Continue following along with all the science from DART, including the latest images and updates on the mission website. Plus, explore even more resources on this handy page.
Teach It
The mission is a great opportunity to engage students in the real world applications of STEM topics. Start exploring these lessons and resources to get students engaging in STEM along with the mission.
Educator Guides
Expert Talks
Student Activities
Articles
- Teachable Moments
How NASA Studies and Tracks Asteroids Near and Far
Here’s how NASA uses math and science to track the movements of asteroids and find out what they’re made of – and students can, too.
- Meet JPL Interns
From Island Life to Spotting Asteroids for NASA
Meet a JPL intern whose journey took her from the remote island of Saipan to a team helping track asteroids at NASA.
Resources for Kids
Check out these related resources for kids from NASA Space Place:
- Article for Kids: Asteroid or Meteor: What's the Difference?
- Article for Kids: What Is an Asteroid?
- Article for Kids: Why Does the Moon Have Craters?
- Article for Kids: What Is an Impact Crater?
Explore More
- Facts & Figures: Didymos In Depth
- Facts & Figures: DART Mission
- Website: DART Mission
- Gallery: DART Mission Images and Videos
- Facts & Figures: Asteroid Watch
- Gallery: Next Five Asteroid Approaches
- Articles: Asteroid News and Images from JPL
- Eyes on Asteroids
- Eyes on the Solar System - DART Impact
- Quiz: Are You a Planetary Defnder?
- Center for Near-Earth Object Studies
TAGS: Asteroids and Comets, DART, near-Earth objects, planetary defense, Science, K-12 Education, Teachers, Educators, Parents, Teachable Moments, Asteroid TM
Meet JPL Interns | July 28, 2022
Interns Explore the Future at NASA-JPL
We talked to a few JPL interns about what they've been working on, how they're taking NASA into the future, and what it all means to them.
Despite the challenges of the past two years, it’s been a busy time for NASA’s Jet Propulsion Laboratory. Among the Lab’s activities have been the launch and landing of a new Mars rover, preparations for sending a spacecraft to explore an ocean world beyond Earth, first light for missions studying our changing climate and the universe beyond, and the development of technology to help address the COVID pandemic.
All the while, JPL interns have continued supporting scientists, engineers, and technologists behind the scenes to make those missions and projects happen.
More than 600 summer interns are taking part in that crucial work – both in-person at the laboratory in Southern California as well as from their homes and dorms across the country. In May, JPL welcomed summer interns back on site for the first time since 2019 while continuing to offer remote internships as projects allow.
We wanted to hear what interns have been up to, how they're contributing to NASA missions and science, and what the experience has meant to them. So we caught up with three students who have helped see the lab through the last year or two – and in one case, seven years. Watch their stories in the video above.
Explore More
-
Apply Now
Discover exciting internships and research opportunities at the leading center for robotic exploration of the solar system.
-
Article: How to Get an Internship at JPL
Here's everything you need to know about the world of JPL internships, the skills that will help you stand out, and how to get on the right trajectory even before college.
- All Audiences
Blog: Meet JPL Interns
Hear stories from interns pushing the boundaries of space exploration and science at the leading center for robotic exploration of the solar system.
- Join the conversation and find out about the latest opportunities with @NASAJPL_Edu on Twitter, Facebook, and Instagram.
- JPL Lecture Series
- JPL Jobs
- JPL Newsletter
- JPL News
- People of NASA
- NASA Internships
- Careers at NASA
The laboratory’s STEM internship and fellowship programs are managed by the JPL Education Office. Extending the NASA Office of STEM Engagement’s reach, JPL Education seeks to create the next generation of scientists, engineers, technologists and space explorers by supporting educators and bringing the excitement of NASA missions and science to learners of all ages.
Career opportunities in STEM and beyond can be found online at jpl.jobs. Learn more about careers and life at JPL on LinkedIn and by following @nasajplcareers on Instagram.
TAGS: Interns, Internships, College Students, Science, Engineering, InSight, Mars, Europa, Ocean Worlds, Enceladus, Saturn, Cassini, Ceres
Teachable Moments | May 12, 2022
Telescopes Get Extraordinary View of Milky Way's Black Hole
Find out how scientists captured the first image of Sagittarius A*, why it's important, and how to turn it into a learning opportunity for students.
Our home galaxy, the Milky Way, has a supermassive black hole at its center, but we’ve never actually seen it – until now. The Event Horizon Telescope, funded by the National Science Foundation, has released the first image of our galactic black hole, Sagittarius A* (pronounced “Sagittarius A-star” and abbreviated Sgr A*).
Read on to find out how the image was acquired and learn more about black holes and Sagittarius A*. Then, explore resources to engage learners in the exciting topic of black holes.
How Black Holes Work
A black hole is a location in space with a gravitational pull so strong that nothing, not even light, can escape it. A black hole’s outer edge, called its event horizon, defines the spherical boundary where the velocity needed to escape exceeds the speed of light. Matter and radiation fall in, but they can’t get out. Because not even light can escape, a black hole is literally black. Contrary to their name’s implication, black holes are not empty. In fact, a black hole contains a great amount of matter packed into a relatively small space. Black holes come in various sizes and can exist throughout space.
We can surmise a lot about the origin of black holes from their size. Scientists know how some types of black holes form, yet the formation of others is a mystery. There are three different types of black holes, categorized by their size: stellar-mass, intermediate-mass, and supermassive black holes.
Stellar-mass black holes are found throughout our Milky Way galaxy and have masses less than about 100 times that of our Sun. They comprise one of the possible endpoints of the lives of high-mass stars. Stars are fueled by the nuclear fusion of hydrogen, which forms helium and other elements deep in their interiors. The outflow of energy from the central regions of the star provides the pressure necessary to keep the star from collapsing under its own weight.
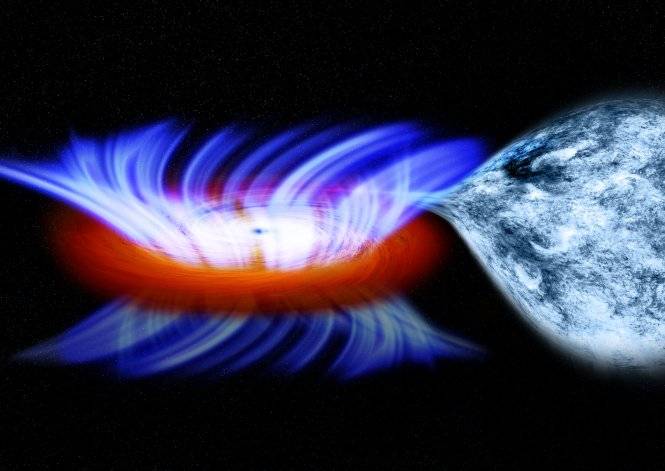
This illustration shows a binary system containing a stellar-mass black hole called IGR J17091-3624. The strong gravity of the black hole, on the left, is pulling gas away from a companion star on the right. This gas forms a disk of hot gas around the black hole, and the wind is driven off this disk. Image credit: NASA/CXC/M.Weiss | › Full image and caption
Once the fuel in the core of a high-mass star has completely burned out, the star collapses, sometimes producing a supernova explosion that releases an enormous amount of energy, detectable across the electromagnetic spectrum. If the star’s mass is more than about 25 times that of our Sun, a stellar-mass black hole can form.
Intermediate-mass black holes have masses between about 100 and 100,000 times that of our Sun. Until recently, the existence of intermediate-mass black holes had only been theorized. NASA’s Chandra X-ray Observatory has identified several intermediate-mass black hole candidates by observing X-rays emitted by the gas surrounding the black hole. The Laser Interferometer Gravitational Wave Observatory, or LIGO, funded by the National Science Foundation, detected the merger of two stellar-mass black holes with masses 65 and 85 times that of our Sun forming an intermediate-mass black hole of 142 solar masses. (Some of the mass was converted to energy and about nine solar masses were radiated away as gravitational waves.)
Supermassive black holes contain between a million and a billion times as much mass as a stellar-mass black hole. Scientists are uncertain how supermassive black holes form, but one theory is that they result from the combining of stellar-mass black holes.

This chart illustrates the relative masses of super-dense cosmic objects, ranging from white dwarfs to the supermassive black holes encased in the cores of most galaxies. | › Full image and caption
Our local galactic center’s black hole, Sagittarius A*, is a supermassive black hole with a mass of about four million suns, which is fairly small for a supermassive black hole. NASA’s Hubble Space Telescope and other telescopes have determined that many galaxies have supermassive black holes at their center.

This image shows the center of the Milky Way galaxy along with a closer view of Sagittarius A*. It was made by combining X-ray images from NASA's Chandra X-ray Observatory (blue) and infrared images from the agency's Hubble Space Telescope (red and yellow). The inset shows Sgr A* in X-rays only, covering a region half a light year wide. Image credit: X-ray: NASA/UMass/D.Wang et al., IR: NASA/STScI | › Full image and caption
Why They're Important
Black holes hold allure for everyone from young children to professional astronomers. For astronomers, in particular, learning about Sagittarius A* is important because it provides insights into the formation of our galaxy and black holes themselves.
Understanding the physics of black hole formation and growth, as well as their surrounding environments, gives us a window into the evolution of galaxies. Though Sagittarius A* is more than 26,000 light years (152 quadrillion miles) away from Earth, it is our closest supermassive black hole. Its formation and physical processes influence our galaxy as galactic matter continually crosses the event horizon, growing the black hole’s mass.
Studying black holes also helps us further understand how space and time interact. As one gets closer to a black hole, the flow of time slows down compared with the flow of time far from the black hole. In fact, according to Einstein’s theory of general relativity, the flow of time slows near any massive object. But it takes an incredibly massive object, such as a black hole, to make an appreciable difference in the flow of time. There's still much to learn about what happens to time and space inside a black hole, so the more we study them, the more we can learn.
How Scientists Imaged Sagittarius A*
Black holes, though invisible to the human eye, can be detected by observing their effects on nearby space and matter. As a result of their enormous mass, black holes have extremely high gravity, which pulls in surrounding material at rapid speeds, causing this material to become very hot and emit X-rays.
This video explains how Sagittarius A* appears to still have the remnants of a blowtorch-like jet dating back several thousand years. Credit: NASA | Watch on YouTube
X-ray-detecting telescopes such as NASA’s Chandra X-ray Observatory can image the material spiraling into a black hole, revealing the black hole’s location. NASA’s Hubble Space Telescope can measure the speed of the gas and stars orbiting a point in space that may be a black hole. Scientists use these measurements of speed to determine the mass of the black hole. Hubble and Chandra are also able to image the effects of gravitational lensing, or the bending of light that results from the gravitational pull of black holes or other high-mass objects such as galaxies.

The thin blue bull's-eye patterns in this Hubble Space Telescope image are called "Einstein rings." The blobs are giant elliptical galaxies roughly 2 to 4 billion light-years away. And the bull's-eye patterns are created as the light from galaxies twice as far away is distorted into circular shapes by the gravity of the giant elliptical galaxies. | › Full image and caption
To directly image the matter surrounding a black hole, thus revealing the silhouette of the black hole itself, scientists from around the world collaborated to create the Event Horizon Telescope. The Event Horizon Telescope harnesses the combined power of numerous telescopes around the world that can detect radio-wave emissions from the sky to create a virtual telescope the size of Earth.
Narrated by Caltech’s Katie Bouman, this video explains how she and her fellow teammates at the Event Horizon Telescope project managed to take a picture of Sagittarius A* (Sgr A*), a beastly black hole lying 27,000 light-years away at the heart of our Milky Way galaxy. Credit: Caltech | Watch on YouTube
In 2019, the team released the first image of a black hole's silhouette when they captured the glowing gasses surrounding the M87* galactic black hole nearly 53 million light-years (318 quintillion miles) away from Earth. The team then announced that one of their next endeavors was to image Sagittarius A*.
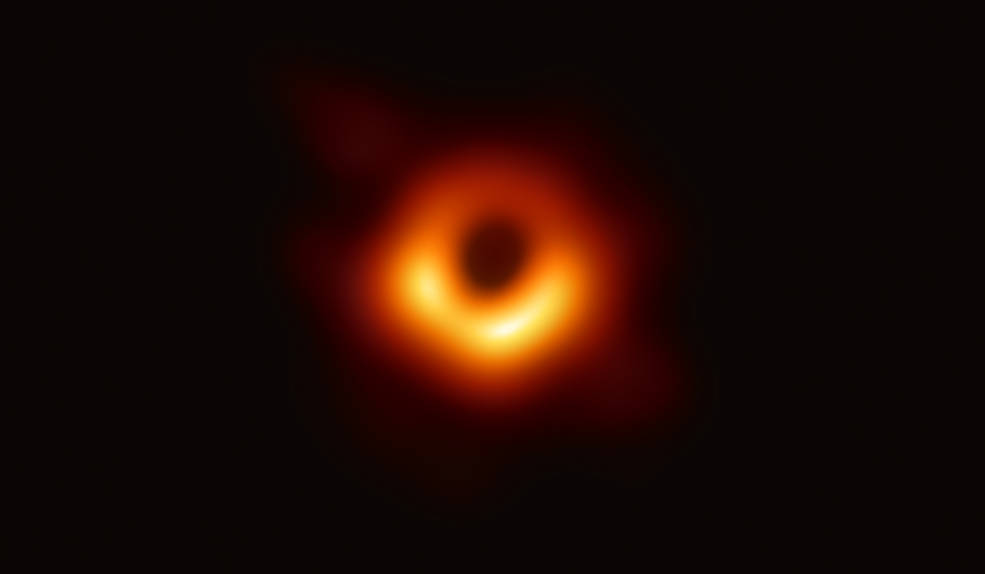
Captured by the Event Horizon Telescope in 2019, this image of the the glowing gasses surrounding the M87* black hole, was the first image ever captured of a black hole. Image credit: Event Horizon Telescope Collaboration | + Expand image
To make the newest observation, the Event Horizon Telescope focused its array of observing platforms on the center of the Milky Way. A telescope array is a group of telescopes arranged so that, as a set, they function similarly to one giant telescope. In addition to the telescopes used to acquire the M87* image, three additional radio telescopes joined the array to acquire the image of Sagittarius A*: the Greenland Telescope, the Kitt Peak 12-meter Telescope in Arizona, and the NOrthern Extended Millimeter Array, or NOEMA, in France.
This image of the center of our Milky Way galaxy representing an area roughly 400 light years across, has been translated into sound. Listen for the different instruments representing the data captured by the Chandra X-ray Observatory, Hubble Space Telescope, and Spitzer Space Telescope. The Hubble data outline energetic regions where stars are being born, while Spitzer's data captures glowing clouds of dust containing complex structures. X-rays from Chandra reveal gas heated to millions of degrees from stellar explosions and outflows from Sagittarius A*. Credit: Chandra X-ray Observatory | Watch on YouTube
The distance from the center of Sagittarius A* to its event horizon, a measurement known as the Schwarzschild radius, is enormous at seven million miles (12,000,000 kilometers or 0.08 astronomical units). But its apparent size when viewed from Earth is tiny because it is so far away. The apparent Schwarzschild radius for Sagittarius A* is 10 microarcseconds, about the angular size of a large blueberry on the Moon.
Acquiring a good image of a large object that appears tiny when viewed from Earth requires a telescope with extraordinarily fine resolution, or the ability to detect the smallest possible details in an image. The better the resolution, the better the image and the more detail the image will show. Even the best individual telescopes or array of telescopes at one location do not have a good enough resolution to image Sagittarius A*.
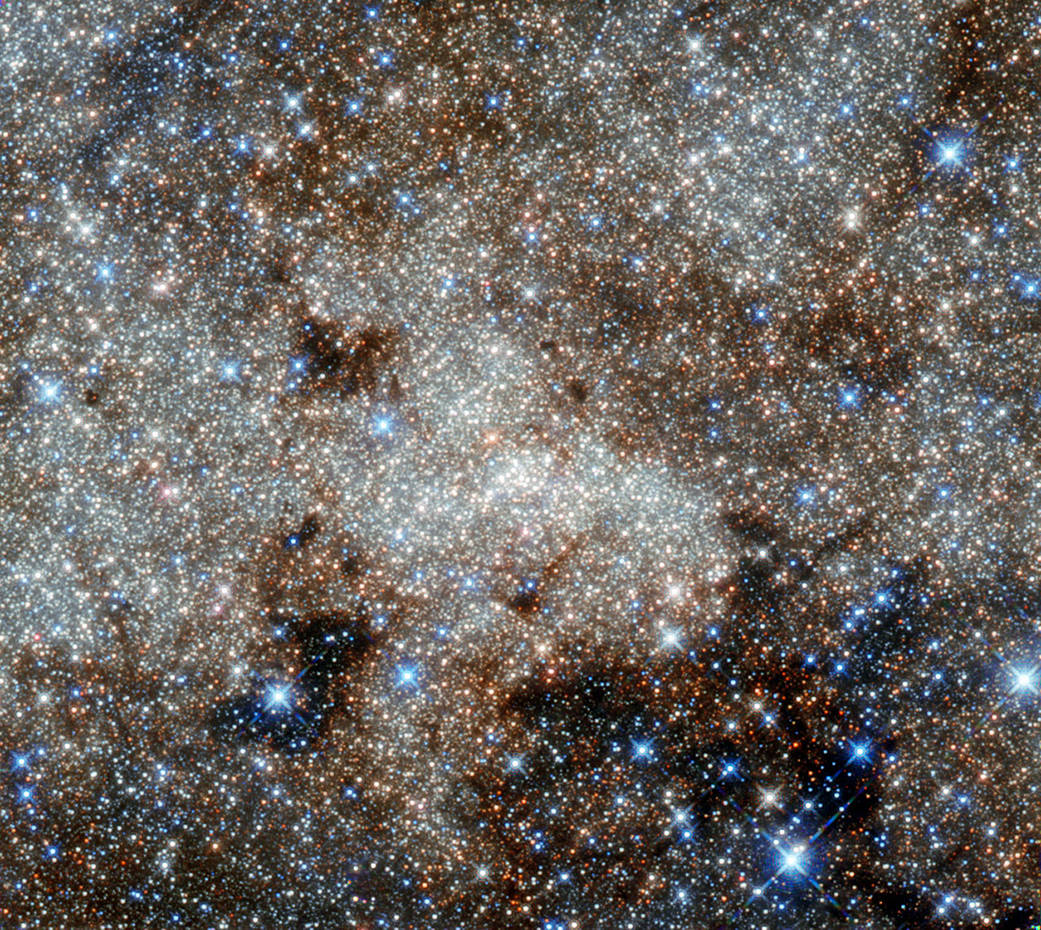
This image captured by NASA's Hubble Space Telescope shows the star-studded center of the Milky Way towards the constellation of Sagittarius. Even though you can't see our galaxy's central black hole directly, you might be able to pinpoint its location based on what you've learned about black holes thusfar. Image credit: NASA, ESA, and G. Brammer | › Full image and caption
The addition of the 12-meter Greenland Telescope, though a relatively small instrument, widened the diameter, or aperture, of the Event Horizon Telescope to nearly the diameter of Earth. And NOEMA – itself an array of twelve 15-meter antennas with maximum separation of 2,500 feet (760 meters) – helped further increase the Event Horizon Telescope’s light-gathering capacity.
Altogether, when combined into the mighty Event Horizon Telescope, the virtual array obtained an image of Sagittarius A* spanning about 50 microarcseconds, or about 1/13th of a billionth the span of the night sky.

Sagittarius A* is more than 26,000 light years (152 quadrillion miles) away from Earth and has the mass of 4 million suns. Image credit: Event Horizon Telescope | › Full image and caption
While the Event Horizon Telescope was busy capturing the stunning radio image of Sagittarius A*, an additional worldwide contingent of astronomical observatories was also focused on the black hole and the region surrounding it. The aim of the team, known as the Event Horizon Telescope Multiwavelength Science Working Group, was to observe the black hole in other parts of the electromagnetic spectrum beyond radio. As part of the effort, X-ray data were collected by NASA’s Chandra X-ray Observatory, Nuclear Spectroscopic Telescope (NuSTAR), and Neil Gehrels Swift Observatory, additional radio data were collected by the East Asian Very Long-Baseline Interferometer (VLBI) network and the Global 3 millimeter VLBI array, and infrared data were collected by the European Southern Observatory’s Very Large Telescope.
The data from these multiple platforms will allow scientists to continue building their understanding of the behavior of Sagittarius A* and to refine their models of black holes in general. The data collected from these multiwavelength observations are crucial to the study of black holes, such as the Chandra data revealing how quickly material falls in toward the disk of hot gas orbiting the black hole’s event horizon. Data such as these will hopefully help scientists better understand black hole accretion, or the process by which black holes grow.
Teach It
Check out these resources to bring the real-life STEM of black holes into your teaching, plus learn about opportunities to involve students in real astronomy research.
-
Educator Guide: Dropping In With Gravitational Waves
Students develop a model to represent the collision of two black holes, the gravitational waves that result and the waves' propagation through spacetime.
Subject Science
Grades 6-8
Time 30-60 mins
-
Teachable Moments: How Scientists Captured the First Image of a Black Hole
Find out how scientists created a virtual telescope as large as Earth itself to capture the first image of a black hole's silhouette.
-
Teachable Moments: Gravitational Waves Detected for the First Time
Find out how researchers proved part of Albert Einstein’s Theory of General Relativity, then create a model of the Nobel Prize-winning experiment in the classroom.
Explore More
Articles
-
Teachable Moments: Learn About the Universe With the James Webb Space Telescope
Get a look into the science and engineering behind the largest and most powerful space telescope ever built while exploring ways to engage learners in the mission.
-
JPL Education ‘Teachable Moment’ Inspires Winning Science Fair Project
A “teachable moment” turned into a science fair win for an eighth-grader in Ontario, Canada, who based his project on a classroom activity from NASA’s Jet Propulsion Laboratory.
Educator Guides
Student Activities
Check out these related resources for students from NASA’s Space Place
Across the NASA-Verse
- Educator Guide: Black Hole Math
- NASA/IPAC TeacherArchive Research Program
- Student Resources: Chandra
- Articles: Hubble - Black Holes
- Audio: Sonification of the Milky Way galactic center
- Audio: Sonification of the M87 black hole
- Interactive: Sagittarius A*
- Videos: Hubble - Black Holes
- Website: NASA Science - Black Holes
- Download: A Galaxy Full of Black Holes Presentation
- Expert Talk: Imaging a Black Hole Lecture
- Website: NASA - Black Holes
- Article: Black Hole Image Makes History
- Graphic: Anatomy of a Black Hole
This Teachable Moment was created in partnership with NASA’s Universe of Learning. Universe of Learning materials are based upon work supported by NASA under award number NNX16AC65A to the Space Telescope Science Institute, working in partnership with Caltech/IPAC, Center for Astrophysics | Harvard & Smithsonian, and the Jet Propulsion Laboratory.
TAGS: Black hole, Milky Way, galaxy, universe, stars, teachers, educators, lessons, Teachable Moments, K-12, science
Teacher Feature | April 25, 2022
Back in the Classroom and Getting Creative With STEAM
This fourth-grade teacher is finding creative ways to get her students back into the flow of classroom learning with the help of STEAM education resources from JPL.
Jackie Prosser is a fourth-grade teacher in Fairfield, California, finishing her second year as a classroom teacher. She is a recent graduate of the University of California, Riverside, where she simultaneously received her teaching credential and her master's in education. This was where I was fortunate enough to meet Miss Prosser, through a collaboration between the Education Office at NASA's Jet Propulsion Laboratory and UCR designed to help new teachers incorporate STEM into their future classrooms. She and her cohort immediately struck me as passionate future teachers already exploring unique ways to bring space science into their teaching.
But it's been a challenging transition for Miss Prosser and teachers like her who started their careers amid a pandemic. She began her student-teaching in person only to find that she would have to switch to teaching remotely just four months into the job. Now, she's back in the classroom but facing new challenges getting students up to speed academically while reacquainting them with the social aspects of in-person learning.
I caught up with her to find out how she's managing the transition and developing creative ways to support the individual needs of her students and, at the same time, incorporating science and art into her curriculum with the help of STEAM resources from the JPL Education Office.
What made you want to become an elementary school teacher?
Originally, I became a teacher because I love to see that moment of light when a concept finally clicks in a kid’s mind. I am still a teacher (even after the craziest two years ever) because every kid deserves someone to fight for them, and I know I can be that person for at least 32 kids a year.
I love to teach young kids especially for two reasons. The first is their honesty; no one will tell you exactly like it is like a nine-year-old will. The second is that I love the excitement kids have for learning at this age.
It has been a bumpy couple years, especially this past school year when it was unclear if we would be remote again or back in the classroom. How has it been coming back from remote learning?
Coming back from remote learning has been an incredible challenge, but we’ve come a long way since the beginning of the year. Students really struggled being back in a highly structured environment. It was very hard to balance meeting the individual needs of each student and getting them used to the structure and expectations of the classroom.
My fourth graders were online for the last part of second grade and a vast majority of third grade. This is when students really start to solve conflicts and regulate their emotions with less support from adults. I have seen a lot more problems with emotion regulation and conflict among my students this year than in years past.
There is a lot of pressure on teachers right now to make up for all the learning loss and for students being behind on grade-level standards. But I don’t think enough people talk about how much joy and social interaction they also lost during remote learning. Teachers are also feeling the pressure of that. I want to help my students be the very best versions of themselves and being happy and comfortable with themselves is a huge part of that.

A student looks at a page from the NASA Solar System Exploration website. Image courtesy: Jackie Prosser | + Expand image
How do you structure your class to get students back in the flow of a school setting?
I use a lot of manipulatives in my math lessons and try to make their learning as hands-on as possible. I also teach math in small groups to be able to better meet the individual needs of my students. I have one group with me learning the lesson, one group doing their independent practice of the skill, and one group on their computers. Then, the students switch until each group has done each activity.
You’re a big fan of science and came to several JPL Education workshops while you were still in school yourself. Are there JPL Education resources that you have found particularly impactful for your students?
I have always loved teaching science. It is so often left behind or pushed aside. I think a lot of time that happens because teachers feel like they do not have enough background knowledge to teach high-quality science lessons or they think that the lessons will add to the already enormous workload teachers have. My district does not have an adopted or prescribed curriculum for teachers to follow, so we have a lot of freedom for when and how to make the time for STEAM.
The education resources [from NASA's Jet Propulsion Laboratory] have made it so easy for me to teach and get kids excited about science, and my kids absolutely love them. Our favorites always seem to be Make a Paper Mars Helicopter and Art and the Cosmic Connection.
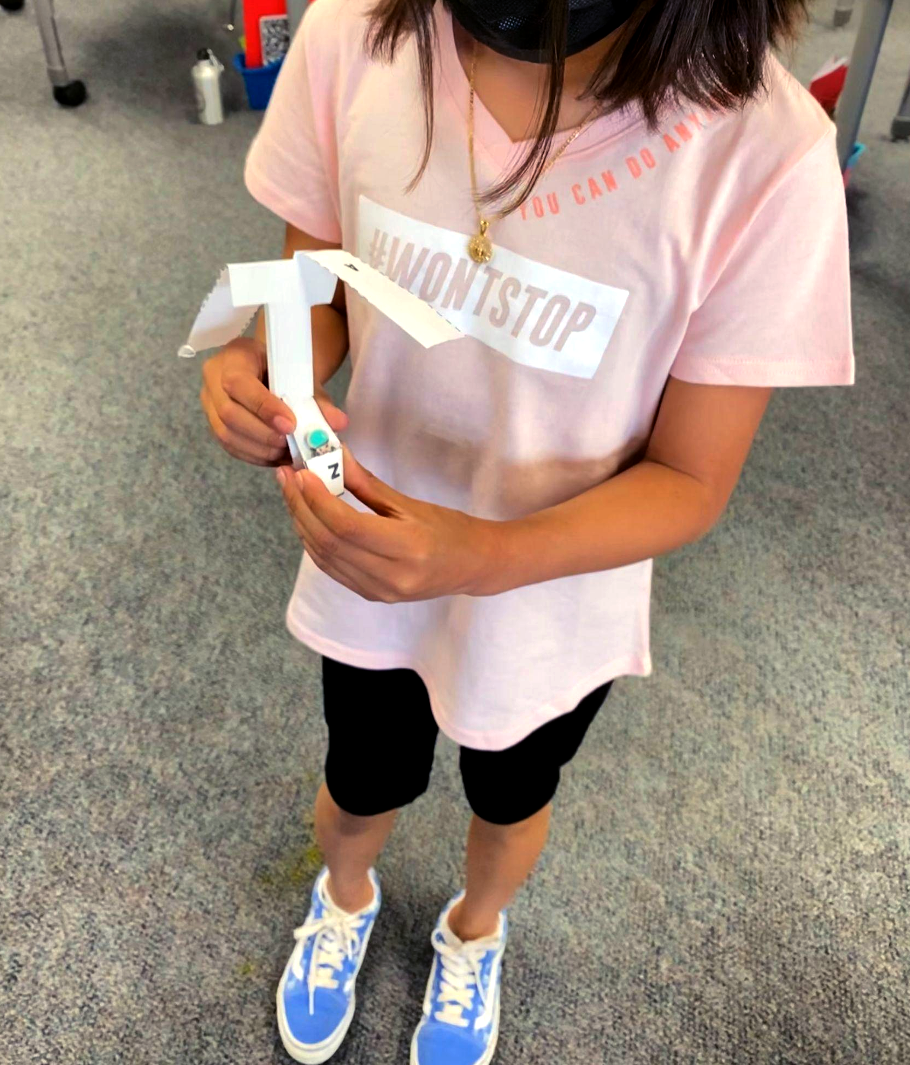
A student holds a paper Mars helicopter. Image courtesy: Jackie Prosser | + Expand image
I also am part of my district’s science pilot program. It has been so cool to be able to decide what curriculum to pilot and watch my students test it out and give feedback on their learning. Last year, I had the amazing opportunity to teach science for two elementary schools’ summer programs. My partner teacher and I got to create the curriculum for them, and we pulled a ton of lessons from the JPL Education website. It was by far the most fun I have ever had at a job.
Despite being a new teacher, you’ve already seen so much. How have you navigated the changing landscape?
I have an amazing network of teachers supporting me at every turn. My grade-level team and my friends from my credential program are some of the most amazing people and educators I have ever met. There is no way I would be able to get through the more difficult aspects of teaching without them.
I am also coaching the boys soccer team, directing the school’s "Lion King Jr." play, contributing to the science pilot program, and serving on the social committee for teachers and staff. I love using these different roles to make connections with not just my students, but also students from all grades.
Looking for ways to bring NASA STEM into your classroom or already have a great idea? The Education Office at NASA's Jet Propulsion Laboratory serves educators in the greater Los Angeles area. Contact us at education@jpl.nasa.gov.
Explore More
-
Make a Paper Mars Helicopter
In this lesson, students build a paper helicopter, then improve the design and compare and measure performance.
Subject Engineering
Grades 2-8
Time 30-60 mins
-
Art and the Cosmic Connection
Students use art to describe and recognize the geology on planetary surfaces.
Subject Science
Grades K-12
Time 1-2 hrs
TAGS: Teachers, School, Remote School, Classroom, Instruction, K-12, Fourth Grade, STEAM, Science, Math, Art, UC Riverside, resources, lessons
Edu News | January 26, 2022
24 STEM Lessons You Can Quickly Deploy in the Classroom
Calling all teachers pressed for time, substitutes looking for classroom activities that don't require a lot of prep, and others hoping to keep students learning in especially chaotic times: We've got a new collection of lessons and activities that you can quickly deploy.
Read on to explore our collection of Quick and Easy STEM lessons and student activities, organized by grade band. Get everything you need to guide students through standards-aligned lessons featuring connections to real NASA missions and science as well as links to student projects, which can be led by teachers or assigned as independent activities.
Grades K-2
-
Make a Paper Mars Helicopter
In this lesson, students build a paper helicopter, then improve the design and compare and measure performance.
Subject Engineering
Grades 2-8
Time 30-60 mins
-
Student Project: Make a Paper Mars Helicopter
Build a paper helicopter, then see if you can improve the design like NASA engineers did when making the first helicopter for Mars.
Subject Engineering
Grades 2-8
Time 30-60 mins
-
What Tools Would You Take to Mars?
Students decide what they want to learn from a robotic mission to Mars and what tools they will put on their robot to accomplish their goals.
Subject Science
Grades K-2
Time 30-60 mins
-
Rockets by Size
Students cut out, color and sequence paper rockets in a simple mathematics lesson on measurement.
Subject Math
Grades K-2
Time 30-60 mins
-
Rocket Math
Students use rocket manipulatives to help them develop number sense, counting, addition and subtraction skills.
Subject Math
Grades K-1
Time 30-60 mins
-
Tangram Rocket
Students use tangrams to create rockets while practicing shape recognition.
Subject Math
Grades K-1
Time 1-2 hrs
-
Student Project: Build a Rover and More With Shapes
Use geometric shapes called tangrams to build a rover and other space-themed designs!
Subject Math
Grades K-2
Time Less than 30 mins
-
Student Project: Build a Rocket and More With Shapes
Use geometric shapes called tangrams to build a rocket and other space-themed designs!
Subject Math
Grades K-2
Time Less than 30 mins
-
Mineral Mystery Experiment
Students explore the science behind an intriguing planetary feature by creating saline solutions and then observing what happens when the solutions evaporate.
Subject Science
Grades 2-12
Time 2 sessions of 30-60 mins
-
Student Project: Do a Mineral Mystery Experiment
Dissolve salts in water, then observe what happens when the water evaporates.
Subject Science
Grades 2-12
Time 2 sessions of 30-60 mins
-
What Do You Know About Mars?
Students decide what they want to learn from a robotic mission to Mars.
Subject Science
Grades K-2
Time Less than 30 mins
-
Melting Ice Experiment
Students make predictions and observations about how ice will melt in different conditions then compare their predictions to results as they make connections to melting glaciers.
Subject Science
Grades 2-12
Time 30-60 mins
-
Parachute Design
Students design and test parachute landing systems to successfully land a probe on target.
Subject Engineering
Grades K-2
Time 1-2 hrs
-
Planetary Poetry
In this cross-curricular STEM and language arts lesson, students learn about planets, stars and space missions and write STEM-inspired poetry to share their knowledge of or inspiration about these topics.
Subject Science
Grades 2-12
Time 1-2 hrs
-
Student Project: Write a Poem About Space
Are you a space poet, and you didn't even know it? Find out how to create your own poems inspired by space!
Subject Science
Grades 2-12
Time 30-60 mins
-
Ocean World: Earth Globe Toss Game
Students use NASA images and a hands-on activity to compare the amounts of land and surface water on our planet.
Subject Science
Grades K-6
Time Less than 30 mins
-
Simple Rocket Science Continued
Students gather data on a balloon rocket launch, then create a simple graph to show the results of the tests.
Subject Math
Grades K-2
Time 30-60 mins
-
Spaghetti Anyone? Building with Pasta
Students use the engineering design process to build a structure to handle the greatest load and gain first-hand experience with compression and tension forces.
Subject Engineering
Grades K-8
Time Less than 30 mins
-
Student Project: Building With Spaghetti
Use spaghetti to build a tower modeled after the giant structures NASA uses to talk to spacecraft.
Subject Engineering
Grades K-8
Time 30-60 mins
-
Simple Rocket Science
Students perform a simple science experiment to learn how a rocket works and demonstrate Newton’s third law of motion.
Subject Science
Grades K-2
Time 30-60 mins
Grades 3-5
-
Make a Paper Mars Helicopter
In this lesson, students build a paper helicopter, then improve the design and compare and measure performance.
Subject Engineering
Grades 2-8
Time 30-60 mins
-
Student Project: Make a Paper Mars Helicopter
Build a paper helicopter, then see if you can improve the design like NASA engineers did when making the first helicopter for Mars.
Subject Engineering
Grades 2-8
Time 30-60 mins
-
Soda-Straw Rockets
Students study rocket stability as they design, construct and launch paper rockets using soda straws.
Subject Engineering
Grades 4-8
Time Less than 30 mins
-
Student Project: Make a Straw Rocket
Create a paper rocket that can be launched from a soda straw – then, modify the design to make the rocket fly farther!
Subject Engineering
Grades 4-8
Time Less than 30 mins
-
Rocket Activity: Heavy Lifting
Students construct balloon-powered rockets to launch the greatest payload possible to the classroom ceiling.
Subject Engineering
Grades 3-8
Time 30-60 mins
-
Design a Robotic Insect
Students design a robotic insect for an extraterrestrial environment, then compare the process to how NASA engineers design robots for extreme environments like Mars.
Subject Science
Grades 3-5
Time 30-60 mins
-
Student Project: Design a Robotic Insect
Design a robotic insect to go to an extreme environment. Then, compare the design process to what NASA engineers do when building robots for Mars!
Subject Science
Grades 3-5
Time 30-60 mins
-
Mineral Mystery Experiment
Students explore the science behind an intriguing planetary feature by creating saline solutions and then observing what happens when the solutions evaporate.
Subject Science
Grades 2-12
Time 2 sessions of 30-60 mins
-
Student Project: Do a Mineral Mystery Experiment
Dissolve salts in water, then observe what happens when the water evaporates.
Subject Science
Grades 2-12
Time 2 sessions of 30-60 mins
-
How Far Away Is Space?
Students use measurement skills to determine the scale distance to space on a map.
Subject Math
Grades 3-7
Time 30-60 mins
-
Student Project: How Far Away Is Space?
Stack coins and use your measurement skills to figure out the scale distance from Earth's surface to space.
Subject Math
Grades 3-7
Time 30-60 mins
-
Melting Ice Experiment
Students make predictions and observations about how ice will melt in different conditions then compare their predictions to results as they make connections to melting glaciers.
Subject Science
Grades 2-12
Time 30-60 mins
-
Planetary Poetry
In this cross-curricular STEM and language arts lesson, students learn about planets, stars and space missions and write STEM-inspired poetry to share their knowledge of or inspiration about these topics.
Subject Science
Grades 2-12
Time 1-2 hrs
-
Student Project: Write a Poem About Space
Are you a space poet, and you didn't even know it? Find out how to create your own poems inspired by space!
Subject Science
Grades 2-12
Time 30-60 mins
-
Planetary Travel Time
Students will compute the approximate travel time to planets in the solar system using different modes of transportation.
Subject Math
Grades 4-6
Time 30-60 mins
-
The Ring Wing Glider
In this simple engineering design lesson, students turn a piece of paper into an aircraft wing and then try to improve upon their design.
Subject Engineering
Grades 3-8
Time 30-60 mins
-
Student Project: Make a Paper Glider
Turn a piece of paper into a glider inspired by a NASA design.
Subject Engineering
Grades 3-8
Time 30-60 mins
-
Ocean World: Earth Globe Toss Game
Students use NASA images and a hands-on activity to compare the amounts of land and surface water on our planet.
Subject Science
Grades K-6
Time Less than 30 mins
-
Spaghetti Anyone? Building with Pasta
Students use the engineering design process to build a structure to handle the greatest load and gain first-hand experience with compression and tension forces.
Subject Engineering
Grades K-8
Time Less than 30 mins
-
Student Project: Building With Spaghetti
Use spaghetti to build a tower modeled after the giant structures NASA uses to talk to spacecraft.
Subject Engineering
Grades K-8
Time 30-60 mins
Grades 6-8
-
Make a Paper Mars Helicopter
In this lesson, students build a paper helicopter, then improve the design and compare and measure performance.
Subject Engineering
Grades 2-8
Time 30-60 mins
-
Student Project: Make a Paper Mars Helicopter
Build a paper helicopter, then see if you can improve the design like NASA engineers did when making the first helicopter for Mars.
Subject Engineering
Grades 2-8
Time 30-60 mins
-
Soda-Straw Rockets
Students study rocket stability as they design, construct and launch paper rockets using soda straws.
Subject Engineering
Grades 4-8
Time Less than 30 mins
-
Student Project: Make a Straw Rocket
Create a paper rocket that can be launched from a soda straw – then, modify the design to make the rocket fly farther!
Subject Engineering
Grades 4-8
Time Less than 30 mins
-
Rocket Activity: Heavy Lifting
Students construct balloon-powered rockets to launch the greatest payload possible to the classroom ceiling.
Subject Engineering
Grades 3-8
Time 30-60 mins
-
Mineral Mystery Experiment
Students explore the science behind an intriguing planetary feature by creating saline solutions and then observing what happens when the solutions evaporate.
Subject Science
Grades 2-12
Time 2 sessions of 30-60 mins
-
Student Project: Do a Mineral Mystery Experiment
Dissolve salts in water, then observe what happens when the water evaporates.
Subject Science
Grades 2-12
Time 2 sessions of 30-60 mins
-
How Far Away Is Space?
Students use measurement skills to determine the scale distance to space on a map.
Subject Math
Grades 3-7
Time 30-60 mins
-
Student Project: How Far Away Is Space?
Stack coins and use your measurement skills to figure out the scale distance from Earth's surface to space.
Subject Math
Grades 3-7
Time 30-60 mins
-
Melting Ice Experiment
Students make predictions and observations about how ice will melt in different conditions then compare their predictions to results as they make connections to melting glaciers.
Subject Science
Grades 2-12
Time 30-60 mins
-
Planetary Poetry
In this cross-curricular STEM and language arts lesson, students learn about planets, stars and space missions and write STEM-inspired poetry to share their knowledge of or inspiration about these topics.
Subject Science
Grades 2-12
Time 1-2 hrs
-
Student Project: Write a Poem About Space
Are you a space poet, and you didn't even know it? Find out how to create your own poems inspired by space!
Subject Science
Grades 2-12
Time 30-60 mins
-
Planetary Travel Time
Students will compute the approximate travel time to planets in the solar system using different modes of transportation.
Subject Math
Grades 4-6
Time 30-60 mins
-
The Ring Wing Glider
In this simple engineering design lesson, students turn a piece of paper into an aircraft wing and then try to improve upon their design.
Subject Engineering
Grades 3-8
Time 30-60 mins
-
Student Project: Make a Paper Glider
Turn a piece of paper into a glider inspired by a NASA design.
Subject Engineering
Grades 3-8
Time 30-60 mins
-
Ocean World: Earth Globe Toss Game
Students use NASA images and a hands-on activity to compare the amounts of land and surface water on our planet.
Subject Science
Grades K-6
Time Less than 30 mins
-
Spaghetti Anyone? Building with Pasta
Students use the engineering design process to build a structure to handle the greatest load and gain first-hand experience with compression and tension forces.
Subject Engineering
Grades K-8
Time Less than 30 mins
-
Student Project: Building With Spaghetti
Use spaghetti to build a tower modeled after the giant structures NASA uses to talk to spacecraft.
Subject Engineering
Grades K-8
Time 30-60 mins
-
How Do We See Dark Matter?
Students will make observations of two containers and identify differences in content, justify their claims and make comparisons to dark matter observations.
Subject Science
Grades 6-12
Time Less than 30 mins
Grades 9-12
-
Mineral Mystery Experiment
Students explore the science behind an intriguing planetary feature by creating saline solutions and then observing what happens when the solutions evaporate.
Subject Science
Grades 2-12
Time 2 sessions of 30-60 mins
-
Student Project: Do a Mineral Mystery Experiment
Dissolve salts in water, then observe what happens when the water evaporates.
Subject Science
Grades 2-12
Time 2 sessions of 30-60 mins
-
Melting Ice Experiment
Students make predictions and observations about how ice will melt in different conditions then compare their predictions to results as they make connections to melting glaciers.
Subject Science
Grades 2-12
Time 30-60 mins
-
Planetary Poetry
In this cross-curricular STEM and language arts lesson, students learn about planets, stars and space missions and write STEM-inspired poetry to share their knowledge of or inspiration about these topics.
Subject Science
Grades 2-12
Time 1-2 hrs
-
Student Project: Write a Poem About Space
Are you a space poet, and you didn't even know it? Find out how to create your own poems inspired by space!
Subject Science
Grades 2-12
Time 30-60 mins
-
Let's Go to Mars! Calculating Launch Windows
Students use advanced algebra concepts to determine the next opportunity to launch a spacecraft to Mars.
Subject Math
Grades 9-12
Time 30-60 mins
-
How Do We See Dark Matter?
Students will make observations of two containers and identify differences in content, justify their claims and make comparisons to dark matter observations.
Subject Science
Grades 6-12
Time Less than 30 mins
Explore More
Find our full collection of more than 250 STEM educator guides and student activities in Teach and Learn.
For games, articles, and more activities from NASA for kids in upper-elementary grades, visit NASA Space Place and NASA Climate Kids.
Explore more educational resources and opportunities for students and educators from NASA STEM Engagement.
TAGS: Lessons, Teachers, Educators, Parents, Substitutes, Activities, Students, Science, Engineering, Quick and Easy